- Department of Genetics, Institute of Biology and Ecology, Faculty of Science, Pavol Jozef Šafárik University in Košice, Košice, Slovakia
In this review we summarize the current knowledge about the changes in Hypericum secondary metabolism induced by biotic/abiotic stressors. It is known that the extreme environmental conditions activate signaling pathways leading to triggering of enzymatic and non-enzymatic defense systems, which stimulate production of secondary metabolites with antioxidant and protective effects. Due to several groups of bioactive compounds including naphthodianthrones, acylphloroglucinols, flavonoids, and phenylpropanes, the world-wide Hypericum perforatum represents a high-value medicinal crop of Hypericum genus, which belongs to the most diverse genera within flowering plants. The summary of the up-to-date knowledge reveals a relationship between the level of defense-related phenolic compounds and interspecific differences in the stress tolerance. The chlorogenic acid, and flavonoids, namely the amentoflavone, quercetin or kaempferol glycosides have been reported as the most defense-related metabolites associated with plant tolerance against stressful environment including temperature, light, and drought, in association with the biotic stimuli resulting from plant-microbe interactions. As an example, the species-specific cold-induced phenolics profiles of 10 Hypericum representatives of different provenances cultured in vitro are illustrated in the case-study. Principal component analysis revealed a relationship between the level of defense-related phenolic compounds and interspecific differences in the stress tolerance indicating a link between the provenance of Hypericum species and inherent mechanisms of cold tolerance. The underlying metabolome alterations along with the changes in the activities of ROS-scavenging enzymes, and non-enzymatic physiological markers are discussed. Given these data it can be anticipated that some Hypericum species native to divergent habitats, with interesting high-value secondary metabolite composition and predicted high tolerance to biotic/abiotic stresses would attract the attention as valuable sources of bioactive compounds for many medicinal purposes.
1. Introduction
To resist the impact of a stressful environment, plants evolved adaptation strategies based either on avoidance or tolerance response (Koch et al., 2016). While the avoidance strategy enables the plant to minimize negative effects of a certain stress, the tolerance mechanism relies on the ability to withstand the unfavorable conditions (Puijalon et al., 2011). In response to bacterial, fungal, and viral diseases, or insect and herbivores attacks plants restrict the pathogen multiplication or minimize the effect of infection, or tissue damage (Pagán and García-Arenal, 2018). Similarly, plants adapt to adverse abiotic conditions like freezing (Körner, 2016), drought (Yang et al., 2020), high salinity (Soliman et al., 2018), or mechanical stress (Puijalon et al., 2011) through the tolerance or avoidance mechanisms.
To reveal the underlying relations between different adaptation strategies, the combined genotypic and phenotypic data are usually applied to characterize the patterns of plant adaptation to local environments (Gao et al., 2021). The biochemical markers including secondary metabolites (SMs), phytohormones, or proteins, along with morphological and anatomical traits like plant shape, leaf structure or area, and physiological parameters, such as chlorophyll content, electrolyte leakage, or water potential are usually used as indicators of the biotic/abiotic stresses (Kosakivska, 2008; Fernandez et al., 2016; Soltabayeva et al., 2021).
Even though the ability of plants to adapt to a certain stress requires a unique response leading to appropriate physiological and metabolic alterations, the overall plant defense is associated with an intensive oxygen metabolism generating reactive oxygen species (ROS) including radicals, especially superoxide anion (O2•-), hydroxyl radical (OH•), and non-radical molecules, such as singlet oxygen (1O2), or hydrogen peroxide (H2O2), as well as reactive nitrogen species (RNS), particularly nitric oxide (NO), and NO-derived molecules (You and Chan, 2015; Sewelam et al., 2016). The reactive molecules are produced in plant tissues as by-products of normal cell metabolism and have a dual role in many biological processes; although the ROS and RNS are associated with oxidative damage of tissues, they act as important signals to activate the stress response (Farnese et al., 2016; Kerchev and Van Breusegem, 2022). Spreading a stress signal from the local tissues to entire plant, the ROS waves were recently referred to be required for plant adaptation to abiotic stresses including light or temperature (Zandalinas et al., 2020).
In relation to stress signalization, the ROS and RNS are involved in promoting the acquired systemic plant response comprising: i) the systemic acquired resistance (SAR) initiated by interactions of cells with pathogens (e.g., viruses, bacteria, or fungi), ii) the systemic acquired acclimation (SAA) to abiotic stimuli, such as extreme temperature, irradiation, osmotic stress, or salinity, and iii) the systemic wound response to mechanical stress induced by various biotic or abiotic stimuli (Mittler and Blumwald, 2015). However, in the complex stress signaling network, many other components including calcium (Ca2+), calmodulin (CaM), G-proteins, the plant hormones, and stress-signal molecules like abscisic acid (ABA), salicylic acid (SA), jasmonic acid (JA), and ethylene, the mitogen-activated protein kinases (MAPKs), and transcription factors (TFs) are involved (Sewelam et al., 2016).
When the overproduction of ROS under stress conditions exceeds the cellular scavenging potential, the oxidative destruction of macromolecules, such as lipids and proteins, negatively impacts cellular metabolism leading to changes of membrane fluidity and ion transfer, loss of enzyme activities, inhibition of protein synthesis, damage of cellular components, and activation of a programmed cell death (Kumari et al., 2021). To minimize the oxidative injury, plants possess enzymatic and non-enzymatic antioxidants (AOXs) (Sharma et al., 2012). The ROS detoxifying proteins, such as superoxide dismutase (SOD), catalase (CAT), and enzymes of ascorbate-glutathione (AsA-GSH) cycle, ascorbate peroxidase (APX) or glutathione reductase (GR), represent the main components of the enzymatic AOXs. The ROS-scavenging enzymes were shown to be involved in maintaining plant homeostasis (Caverzan et al., 2016), as well as in ROS signaling (Miller et al., 2010). For example, the deficiency in enzymatic AOXs leads to overproduction of radical molecules, which in turn act as the signal enhancing the plant tolerance to a certain stress (Miller et al., 2007; Vanderauwera et al., 2011; Sachdev et al., 2021). Non-enzymatic antioxidants include the products of plant secondary metabolism, like phenolic compounds, alkaloids, carotenoids, tocopherols, along with small-molecules, such as ascorbate (AsA), glutathione (γ-glutamyl-cysteinyl-glycine, GSH), proline and other amino acids (Latowski et al., 2014; Ashraf et al., 2019; Jan et al., 2021a). The physiological function of SMs in defense responses against abiotic stresses and pathogens, as well as mediation of the interactions between a plant and other organisms, is well documented (Fang et al., 2011; Li et al., 2020; Pang et al., 2021). The accumulation of SMs with antioxidant activities enhance the overall stress tolerance; it has been shown that plants, which are tolerant to oxidative stress exhibit also tolerance to various environmental conditions, such as extreme temperature, drought, salinity, pathogens, and combinations of them (Sewelam et al., 2014; Nguyen et al., 2018; Nadarajah, 2020).
Within the extremely diverse genus Hypericum comprising nearly 500 species, the St. John's wort – Hypericum perforatum – represents the most renowned and investigated species (Coste et al., 2021). H. perforatum is a medicinal plant traditionally used for treatments of depression and other neurological disorders. The Hyperici herba contains a lot of biologically active constituents; phenolic compounds including naphthodianthrones (hypericin and pseudohypericin), phloroglucinol derivatives (hyperforin, adhyperforin), and flavonoids, representing well-known and frequently analyzed SMs. Showing a range of bioactivities, the phenolic compounds were distinguished as valuable phytochemicals for a broad spectrum of pharmaceutical applications (Agapouda et al., 2019). In the modern medicine, hypericins are promising photosensitizers applied in photodynamic diagnosis and therapy of cancer (Kleemann et al., 2014; Theodossiou et al., 2017). Due to inhibitory effect on the growth of microorganisms, the naphthodianthrone compounds are also effective in plant protection against a number of pathogenes (Zambounis et al., 2020; Sytar et al., 2021). The polycyclic polyprenylated acylphloroglucinols (PPAPs) are responsible for most of H. perforatum biological activities, including antidepressant, anti-inflammatory, and antimicrobial effects (Bridi et al., 2018). Flavonoids, especially the flavonols quercetin, kaempferol and their glycosides, contribute to antioxidant properties of H. perforatum alcoholic extract (Makarova et al., 2021). Melatonin (N-acetyl-5-methoxytryptamine) is other important bioactive compound of H. perforatum (Zhou et al., 2021), which has many physiological functions in humans. This molecule is involved in the sleep-wake cycle or the circadian rhythm regulation (Zisapel, 2018), interacts with the immune system (Ma et al., 2020), protects an organism against oxidative stress (Manchester et al., 2015; Galano and Reiter, 2018), exhibits the antidepressant effects (Wang et al., 2022), and is involved in the nerve regeneration processes (Stazi et al., 2021).
Based on the state of the art we discuss the alterations of Hypericum spp. phenolics content in relation to various abiotic and biotic stresses (section 2) with an emphasis on determination of the predictive value of phenolic compounds and other AOXs in revealing the freezing resistance strategy of Hypericum plants which is illustrated in the case study (section 3). We show that phenotyping of phenolics composition, especially flavonoids might contribute to revealing the freezing tolerance/avoidance strategy in the genus Hypericum. As summarized in section 4, several conclusions were made regarding the changes in Hypericum secondary metabolism in relation to overall stress tolerance.
2 Phenotyping of secondary metabolism in response to biotic/abiotic stresses in Hypericum spp.
The genetically predetermined plant metabolite profile is modulated by various external and internal stimuli. Usually, the accumulation of SMs is related to the plant developmental stage and often requires the presence of specialized cells or cell structures (Isah, 2019). The phytochemical composition also depends on the crosstalk between plant and its microbiome (Pang et al., 2021), and is modified by environmental conditions like the intensity of light, extreme temperatures, drought, or salinity (Yang et al., 2018; Wasternack and Strnad, 2019; Li et al., 2020). The stress-induced metabolic alterations are commonly used for studying the function of SMs in plant stress responses (Szymański et al., 2020; Dussarrat et al., 2022). To reveal the stress adaptation strategy, the identification of metabolites that can distinguish between the tolerant and sensitive species is commonly applied (Hall et al., 2022). Based on the monitoring of metabolic markers with predictive value, and their unique combinations, the phenotyping provides a powerful tool for estimation of the extent of plant stress tolerance (Yadav et al., 2019).
In response to external stimuli, several fold increase of phenolic compounds was documented in Hypericum spp. culture systems like in vitro shoot cultures, cell suspensions or greenhouse-grown plants. Various biotic/abiotic stimuli (elicitors) were shown to redirect metabolism toward the enhanced accumulation of SMs including naphthodianthrones, acylphloroglucinols, xanthones, flavonoids, hydroxycinnamic acids, and other metabolites. Up to date, the elicitation potential of a broad spectrum of factors affecting the SMs accumulation was studied: i) herbivores (Sirvent et al., 2003); ii) microorganisms including endophytic fungi (Gadzovska-Simic et al., 2015b; Bálintová et al., 2019), pathogenic fungi (Sirvent and Gibson, 2002; Çirak et al., 2005; Gadzovska-Simic et al., 2012; Meirelles et al., 2013), or bacteria (Pavlík et al., 2007; Tusevski et al., 2017; Bálintová et al., 2019); iii) chemical elicitors, such as stress-signal molecules and growth regulators (Gadzovska et al., 2007; Pavlík et al., 2007; Coste et al., 2011; Gadzovska et al., 2013; Wang et al., 2015), simple sugars, or complex plant carbohydrates (Kirakosyan et al., 2000; Pavlík et al., 2007; Gadzovska-Simic et al., 2014; Gadzovska-Simic et al., 2015a; Bálintová et al., 2019), nanoparticles (Sharafi et al., 2013; Jafarirad et al., 2021); and iv) environmental abiotic stimuli including drought, irradiation, or temperature (Southwell and Bourke, 2001; Zobayed et al., 2006; Sooriamuthu et al., 2013). These results are summarized in Supplementary Table 1 (Supplementary Material S.M.1).
2.1 Naphthodianthrones and acylphloroglucinols
Within the plant kingdom, the polyketide pathway-derived naphthodianthrones are synthesized essentially by some Hypericum spp. Among prenylated polyketides identified in the representatives of Guttiferae (Clusiaceae), the acylphloroglucinols with prenylation patterns are commonly isolated from the genus Hypericum (Crockett and Robson, 2011; Porzel et al., 2014).
The accumulation of naphthodianthrones is restricted to the glandular structures, so-called dark nodules, which are found in approximately 2/3 of Hypericum sections (Ciccarelli et al., 2001; Crockett and Robson, 2011). New mass spectrometry imaging (MSI)-based techniques confirmed co-localization of hypericin, pseudohypericin and their protoforms protohypericin and protopseudohypericin in the dark nodules, as the sites of their accumulation within the leaf. The naphthodianthrones are co-localized in these structures even with other anthraquinones and bisanthraquinones. Similarly, hyperforin, together with its analogues adhyperforin or hyperfirin, were co-localized in the translucent pale cavities (Kusari et al., 2015; Kucharíková et al., 2016; Rizzo et al., 2019; Revuru et al., 2020). Since only members of the sections belonging to clade core Hypericum produce hypericins (Nürk et al., 2013), the application of naphthodianthrones as potential stress-induced markers in metabolic phenotyping is limited. Nevertheless, numerous studies have been focused on improvement of the Hypericum spp. chemical composition by stimulating biosynthesis of hypericins and acylphloroglucinol derivatives using different biotic/abiotic elicitors.
In Hypericum plants, the increased accumulation of hypericins and hyperforin usually signalizes stressful environmental conditions associated with higher altitudes, including the intensity and quality of light, extreme temperatures, or temperature fluctuations (Zobayed et al., 2005; Odabas et al., 2009; Germ et al., 2010; Brechner et al., 2011; Rahnavard et al., 2012). For example, each 70 to 100 µmol m-2 s-1 PAR (photosynthetically active radiation) resulted in a 1.5-fold increase of hypericin content in H. perforatum shoot cultures (Briskin and Gawienowski, 2001). In greenhouse-grown H. perforatum plants, increasing light intensities from 803.4 to 1618.6 µmol m-2 s-1 stimulated continuous rise of hyperforin, hypericin and pseudohypericin contents (Odabas et al., 2009). In relation to light quality, the red light and UV-B stimulated the production of hypericin, pseudohypericin and hyperforin in H. perforatum and H. retusum (Nishimura et al., 2007; Brechner et al., 2011; Namli et al., 2014).
Under higher temperature stress, the ambiguous plant responses were reported. In most of the studies aimed at H. perforatum, the naphthodianthrone and hyperforin content was found to be positively influenced by increasing temperature up to 30 or 35°C (Couceiro et al., 2006; Zobayed et al., 2006; Odabas et al., 2009). On the other side, Yao et al. (2019) and Couceiro et al. (2006) did not observe any stimulatory effect of increasing temperature on either hypericin or hyperforin accumulation in this species. However, accumulation of these compounds differs in plant organs and age of culture. The content of hypericins and hyperforin was the highest at 35°C in the shoots, while in the flowers, the maximum was reached at 20°C or 25°C (Zobayed et al., 2005).
In response to low temperatures, the accumulation of hypericins predominantly depends on a cold stimulus. While the exposure of H. perforatum plants to low but above zero temperature was accompanied by unchanged or significantly lower amount of hypericins, the subfreezing temperature (-4°C) resulted in a 1.6-fold increase of hypericin content (Petijová et al., 2014; Bruňáková et al., 2015). In post-cryogenic regenerants of H. perforatum, H. rumeliacum and H. tetrapterum, more than a 3-fold increase of hypericin accumulation was documented and a remarkable 38-fold increase of hyperforin content was observed in H. rumeliacum (Petijová et al., 2014; Bruňáková and Čellárová, 2017). The stimulatory effect of cryogenic treatment can be appended to its complex abiotic stress nature comprising the dehydration of cells, crystallization of free water, mechanical wounds caused by ice particles and cold itself. However, accumulation of hypericins and phloroglucinols was negatively correlated with a single stressor represented by the acute drought or osmotic stress (Gray et al., 2003; Zobayed et al., 2003).
Relatively low stimulation of naphthodianthrones biosynthesis reaching 1.3 to 4-fold increase was seen in Hypericum spp. shoot cultures subjected to various chemical elicitors. In H. perforatum and H. adenotrichum, the elevated production of hypericins was achieved by the addition of polyethylene glycol (PEG) or sucrose to culture media (Pavlík et al., 2007; Yamaner and Erdag, 2013), and more than 3-fold increase of hypericins was reported in nanoperlite-treated H. perforatum shoot cultures (Jafarirad et al., 2021). In reaction to phytohormones, the accumulation of naphthodianthrones depended on the type, combination, and concentration of phytohormones including the adenine-type (BAP, 6-benzylaminopurine; Kin, kinetin; ZT, zeatin), or phenylurea-type (TDZ; thidiazuron) cytokinins (Liu et al., 2007; Karakas et al., 2009; Coste et al., 2011). The hypericins content also increased when the stress-signaling molecules salicylic acid (SA), jasmonic acid (JA) and methyl-jasmonate (MeJA) were used for elicitation of Hypericum shoot cultures (Sirvent and Gibson, 2002; Liu et al., 2007; Pavlík et al., 2007; Coste et al., 2011; Gadzovska et al., 2013).
For stimulation of plant secondary metabolism by the elicitors of biotic origin, pathogenic or symbiotic microorganisms are usually inactivated, and a mixture of lipopolysaccharides, peptidoglycans and other cell wall components are used. The stimulatory effects of biotic elicitors depend on the type, intensity, and duration of stimuli. The accumulation of naphthodianthrones increased in Hypericum shoots treated with pectin or dextran (Gadzovska-Simic et al., 2014), mannan, pectin and β-1,3-glucan (Kirakosyan et al., 2000; Yamaner and Erdag, 2013). The elicitors derived from endophytic fungal mycelia of Thielavia subthermophila, Piriformospora indica, Fusarium oxysporum and Trichoderma crassum induced a 1.5-fold increase of hypericins in several representatives of the sections Hypericum and Oligostema regardless the elicitor type, while a 2.3-fold higher accumulation of phloroglucinols was observed in H. kouytchense after the treatment with P. indica (Bálintová et al., 2019). The content of phenolic compounds including hypericins and hyperforin also increased upon inoculation of Hypericum plants with pathogenic fungi, such as Colletotrichum gloeosporioides (Sirvent and Gibson, 2002), Diploceras hypericinum, Phytophthora capsici (Çirak et al., 2005), or Nomuraea rileyi (Meirelles et al., 2013). In contrast to unchanged hyperforin content, higher amounts of hypericins were detected in H. perforatum seedlings infected with a mix of arbuscular mycorrhizal fungi (Zubek et al., 2012; Lazzara et al., 2017). The content of naphthodianthrones in Hypericum plants was also stimulated by inoculation with bacteria, for example the bacterial strains isolated from rhizosphere of Nicotiana glauca wild population (Mañero et al., 2012). The inactivated Agrobacterium tumefaciens culture promoted production of hyperforin in H. perforatum shoots, although hypericin accumulation declined (Pavlík et al., 2007). In the transgenic plants of H. tomentosum regenerated form hairy root cultures induced by A. rhizogenes-mediated transformation, higher content of hypericins in comparison with control plants was observed (Henzelyová and Čellárová, 2018). Similarly, higher amount of hypericins along with a 7-fold increase of hyperforin was observed in transgenic lines of H. perforatum plants (Tusevski et al., 2017). Additionally, hypericins and hyperforin content increased upon feeding with herbivores represented by the generalist feeders Spilosoma virginica and S. congrua, or Spodoptera exigua (Sirvent et al., 2003).
Being an integral part of acquired systemic plant responses in Hypericum spp., the increased accumulation of naphthodianthrones and acylphloroglucinols was shown to be linked with various biotic and abiotic stress stimuli. However, applying phototoxic pigments hypericin and pseudohypericin to examine a stress tolerance is limited as the accumulation can only be stimulated to a certain physiological level. When compared with untreated Hypericum plants, the relatively low increase of hypericins content, usually not exceeding a 5-fold, could be attributed to morpho-anatomical structure of the tissues, in which these compounds accumulate. Based on the morphometric leaf parameters of 12 Hypericum species, a cubic degree polynomial regression function was proposed for estimation of the biosynthetic capacity of Hypericum shoot cultures (Kimáková et al., 2018). Furthermore, Saffariha et al. (2021) designed several models to predict changes of hypericin content in relation to ecological and phenological factors.
2.2 Flavonoids and other phenolic compounds
Within plant polyphenols, flavonoids are the best-known metabolites with antioxidant properties. Through the inhibition of ROS generation, scavenging of free radicals, absorption of UV-wavelengths, and protection of cell membranes, these compounds have a fundamental role in plant photoprotection. Moreover, flavonoids were found to act as signal molecules involved in plant developmental processes, including the morphogenetic responses induced by various biotic/abiotic stimuli (Ferdinando et al., 2012; Brunetti et al., 2013; Petrussa et al., 2013; Chandran et al., 2019; DeLuna et al., 2020). Based on distinct substitutions in the basic skeleton containing three phenolic rings, namely two 6-carbon rings A and B linked by the central 3-carbon C ring, the flavonoids can be subdivided into several major subgroups including flavonols, flavones, flavanones, flavanonols, flavanols, anthocyanins, isoflavonoids and chalcones (Panche et al., 2016). Flavonoids are often linked to mono- or oligosaccharides (glucose, galactose, rhamnose, xylose, arabinose) and can be mono-, di-, or tri-glycosylated (Nabavi et al., 2020). Due to a great antioxidant capacity and photoprotection, the flavonols represented by dihydroxy B-ring-substituted quercetin-3-O-glycosides along with monohydroxy B-ring-substituted kaempferol-3-O-glycosides, are the most studied flavonoids (Shah and Smith, 2020).
In relation to environmental conditions, the phytochemical profiling revealed substantial differences in the accumulation of polyphenolic compounds, especially flavonoids and hydroxycinnamates (Tattini et al., 2004). In common, the enhanced accumulation of flavonols and anthocyanins usually indicates a stress exposure (Jan et al., 2021b). Based on the spectrum and abundance of stress-induced metabolites, the flavonoid profile can be used to distinguish between the stress-exposed plants and their unstressed counterparts. For example, the quercetin-3-O-glucuronide/glucoside and kaempferol-3-O-galactoside/glucoside represent specific metabolites displaying significantly higher relative content in water-deficient plants of Vitis vinifera when compared with the unstressed control group (Gago et al., 2017). Furthermore, the species-specific responses to abiotic stress including differences in the content of quercetin and its glycosides enable to distinguish even between related species like Crataegus laevigata and Crataegus monogyna (Kirakosyan et al., 2004). The variation of biosynthetically related metabolites is usually further modified by other abiotic stimuli (de Abreu and Mazzafera, 2005) or concomitant biotic stresses (Schenke et al., 2019). As an example, the variation of quercetin and rutin in water stressed Hypericum brasiliense plants depended on the temperature regime (de Abreu and Mazzafera, 2005).
In the genus Hypericum, flavonoids and hydroxycinnamic acids were shown to be the most prevalent phenolic compounds involved in antioxidant responses (Zdunić et al., 2017). The production of several flavonoid compounds and chlorogenic acid increased in H. perforatum adventitious roots cultured in media supplemented up to 70 g L-1 of sucrose resulting in osmotic stress (Cui et al., 2010); the levels of quercetin aglycone and its glycoside rutin increased in shoots and roots of water-stressed H. brasiliense (de Abreu and Mazzafera, 2005); and the acute drought stress applied on H. perforatum shoots resulted in higher accumulation of quercetin glycosides in floral tissues (Gray et al., 2003). The content of several flavonoids including quercetin, hyperoside (quercetin-3-D-galactoside), rutin (quercetin-3-rutinoside), and quercitrin (quercetin-3-L-rhamnoside), along with kaempferol, amentoflavone, apigenin-7-glucoside, and chlorogenic acid also raised with the increasing light intensity and temperature; based on the relationship between the culture conditions (e.g. temperature, light intensity) and phenolics accumulation, a mathematical model was proposed for the prediction of phenolics content in H. perforatum plants (Odabas et al., 2010).
A visible tissue coloring caused by the enhanced accumulation of flavonoids is usually taken as general indicator of physiological status of a stressed plant (Lu et al., 2017). Sooriamuthu et al. (2013) observed a 3 to 5-fold elevation of flavonoids and anthocyanins in photoinduced reddish-colored plantlets after transferring the etiolated Hypericum hookerianum shoots to the light. In H. perforatum shoot cultures, the reddening of plant organs due to accumulation of anthocyanins signalized the increased osmotic strength of culture medium after addition of sucrose (Pavlík et al., 2007). In our previous research (Bruňáková et al., 2011; Bruňáková and Čellárová, 2016), a purple pigmentation of leaf tissues was also seen in the post-cryogenic regenerants of H. perforatum (Figure 1).
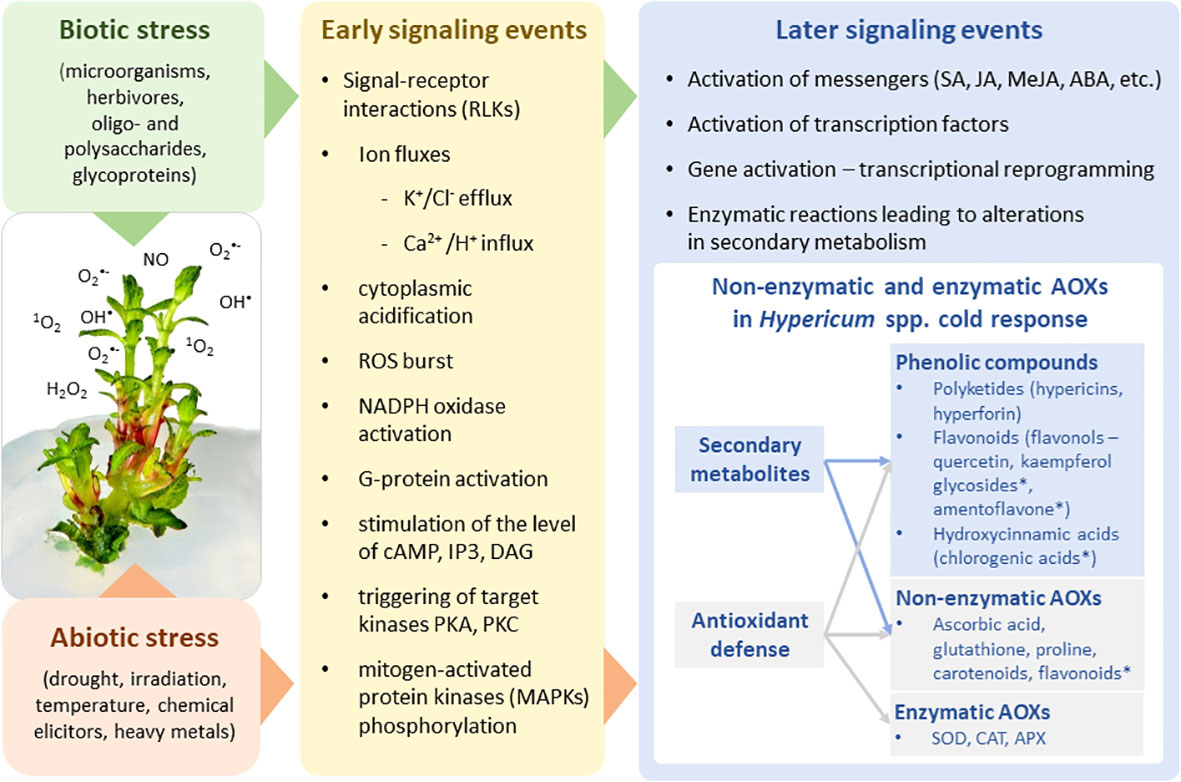
Figure 1 The biotic/abiotic stress signaling pathways comprising the stress perception, signal transduction, and expression of specific stress-related genes resulting in modulation of plant primary and secondary metabolism. Metabolites highlighted by an asterisk are the markers with predictive value in cold-stress response of Hypericum species.
The flavonoids and other phenolic compounds have been shown to strengthen the tolerance to a wide range of elicitors of biotic origin, including the pest and pathogen attacks. In our previous work, the changes in metabolic profiles of Hypericum spp. induced by glucose, starch, chitosan, and elicitors derived from H. perforatum-borne endophytic fungi were shown to be species-specific, depending on the type and intensity of stress stimuli (Bálintová et al., 2019). The content of rutin, hyperoside, isoquercetin (quercetin-3-glucoside) and quercitrin was elevated in all species regardless the elicitor, showing a maximum of 13-fold increase of isoquercetin in H. monogynum shoots treated with P. indica-derived elicitors in the presence of chitosan. On the other hand, the accumulation of amentoflavone and chlorogenic acid depended on the elicitation treatment and Hypericum species; the amentoflavone content raised maximally 15.7-times in H. humifusum shoots after culturing them on media supplemented with potato-dextrose broth (PDB), and a 13-fold increase was detected in H. tetrapterum after the treatment with elicitors derived from T. subthermophila, or A. rhizogenes. A maximum of 31.7-fold elevation of the chlorogenic acid was documented in H. maculatum shoots treated with D-glucose. Almost a 15-fold elevation of the chlorogenic acid was seen after the chitosan treatment in H. kouytchense shoots and a 11.5-fold increase of this metabolite was observed in H. maculatum treated by A. rhizogenes-derived elicitors (Bálintová et al., 2019).
The elevated accumulation of flavonols, hydroxycinnamic acids, xanthones, and other phenolic compounds stimulated by (poly)saccharides in in vitro cultures of Hypericum spp. proves the universal role of saccharides-based elicitation (Kirakosyan et al., 2000; Gadzovska-Simic et al., 2014; Gadzovska-Simic et al., 2015a). The elicitors derived from Aspergillus niger and F. oxysporum stimulated the biosynthesis of phenylpropanoids or flavonoid glycosides in several Hypericum spp. (Azeez and Ibrahim, 2013; Gadzovska-Simic et al., 2015b). Similarly, Franklin et al. (2009), and Tusevski et al. (2015) documented a significant elevation of xanthone biosynthesis in H. perforatum cell cultures stimulated by elicitors derived from A. rhizogenes and A. tumefaciens. An enhanced content of lignin and flavonoids was observed in H. perforatum cell wall biomass after A. tumefaciens elicitation (Singh et al., 2014). In common, the structural components of the cell wall of fungal or bacterial pathogens induce defense reactions in both, the intact plants or plant cell cultures via production of ROS, hypersensitive response, or production of SMs with antimicrobial effects (Angelova et al., 2006).
Melatonin represents another stress-responsive metabolite involved in antioxidant defense in several Hypericum representatives, e.g., H. perforatum, or H. kouytchense (Murch and Saxena, 2006; Nguyen et al., 2018; Chung and Deng, 2020; Khan et al., 2020). According to Zhou et al. (2021), the overexpression of HpSNAT1 gene coding for serotonin N-acetyltransferase (SNAT), the key enzyme involved in melatonin biosynthesis resulted in more than 4-fold increase of melatonin content in response to high salt and drought treatment. In plants, melatonin was shown to contribute to the acquisition of tolerance against various abiotic/biotic stresses including cold (Han et al., 2017; Qari et al., 2022), or pathogen attacks (Lee et al., 2014).
To differentiate between stress tolerant and sensitive plants by metabolic phenotyping, the metabolite alterations induced by biotic/abiotic stimuli should be distinguished from a great naturally existing interspecific variability within this genus (Bálintová et al., 2019). Across the Hypericum spp., the spectrum and abundancy of SMs vary at the population, species and genotype levels (Çirak et al., 2008; Nogueira et al., 2008; Crockett and Robson, 2011; Çirak et al., 2014; Çirak et al., 2015; Çirak et al., 2016; Çirak and Radusiene, 2019; Carrubba et al., 2021), and usually changes during ontogenesis (de Abreu et al., 2004). Recent metabolomics studies reveal both the common and unique alterations in metabolic profiles of plants exposed to biotic/abiotic stresses related to polyphenolic compounds represented by chlorogenic acid, and flavonoids, namely the amentoflavone, quercetin or kaempferol glycosides.
3 Tolerance of Hypericum spp. to cold stress - the case study
The ability to withstand low temperatures represents a limiting factor of geographical distribution of the plant species. Plants adapt to under-zero temperatures through two main adaptation strategies based either on the tolerance, or avoidance responses. The ‘freezing tolerant’ (FT) species tolerate the extracellular ice formation that is accompanied by the dehydration stress; on the other side, the ‘freezing sensitive’ (FS) species prevent the formation of ice crystals in their tissues (Squeo et al., 1991; Sung et al., 2003). While the avoidance strategy predominantly relies on physical adaptations like lowering of the freezing point or supercooling, the tolerance mechanism is mainly associated with metabolic adaptations (Gusta and Wisniewski, 2013). In addition to this, the subjection of FT plants to low, but non-freezing temperatures, increases their ability to survive the otherwise lethal temperatures (below 0°C) through the process known as cold acclimation (Thomashow, 1999; Zandalinas et al., 2020). In FT species, the low but above-zero temperatures induce the higher resistance to freezing based on maintaining the functional photosynthetic apparatus and integrity of cell membranes (Ding et al., 2019; Pan et al., 2022).
To reveal the mechanism, by which the plants resist a potential freezing injury, the combined morphological, physiological, and biochemical data are widely applied. Within the genus Hypericum, several approaches were successfully applied to distinguish between FT and FS species, including the measurement of thermal properties, e.g. the freezing temperature of the leaves, the quantification of the extent of membrane disintegration, e.g. using the electrolyte leakage assay (LT50 values) (Petijová et al., 2014; Bruňáková et al., 2015), or the antioxidant profiling (Skyba et al., 2010; Danova et al., 2012). The changes of plant habitus, mesophyll parenchyma thickness, and chloroplast ultrastructure helped to find an association between the extent of freezing-induced tissue damage and predicted tolerance strategy in post-cryogenic regenerants of several Hypericum representatives (Georgieva et al., 2014; Stoyanova-Koleva et al., 2015).
One of the aims of the case study was to identify the profiling metabolites indicating the freezing tolerance strategy in 10 Hypericum species differing in their geographical distribution, and taxonomically classified to the sections Ascyreia, Androsaemum, Bupleuroides, Hypericum, Oligostema, Myriandra, Webbia and Adenosepalum (Table 1). The phenolic compounds, along with other non-enzymatic antioxidants, proline and carotenoids, as well as enzymatic activities of ROS-scavenging enzymes, superoxide dismutase (SOD), catalase (CAT) and ascorbate peroxidase (APX), were determined following the exposure of plants to 4°C (Bul'ková, 2018). Briefly, Hypericum plants cultured in vitro were used for comparative analyses of untreated (control) plants growing at 23 ± 2°C and plants, which were subjected to 4°C for 7 days. In the extracts of aerial plant parts, the content of phenolic compounds was analyzed by high-performance liquid chromatography (HPLC-DAD); the assessment of non-enzymatic and enzymatic antioxidant systems was done by spectrophotometric methods. Interspecific differences in phenolic composition as well as enzymatic and non-enzymatic AOXs related to cold response were evaluated by the principal component analysis (PCA) and hierarchical cluster analysis (HCA) (Supplementary Material S.M.2).
3.1 Metabolic profiling of plants exposed to 4°C
In control plants, the PCA revealed a great interspecific variability of Hypericum phenolic composition related to anthraquinones, acylphloroglucinol derivatives, flavonoids and chlorogenic acid (Figures 2A, B). According to the determinant metabolites, Hypericum representatives were distributed to two relatively compact groups and a singleton. The HCA-based heatmap (Figure 2C) was used to visualize the differences in the number of phenolic compounds between control plants and plants exposed to the cold treatment.
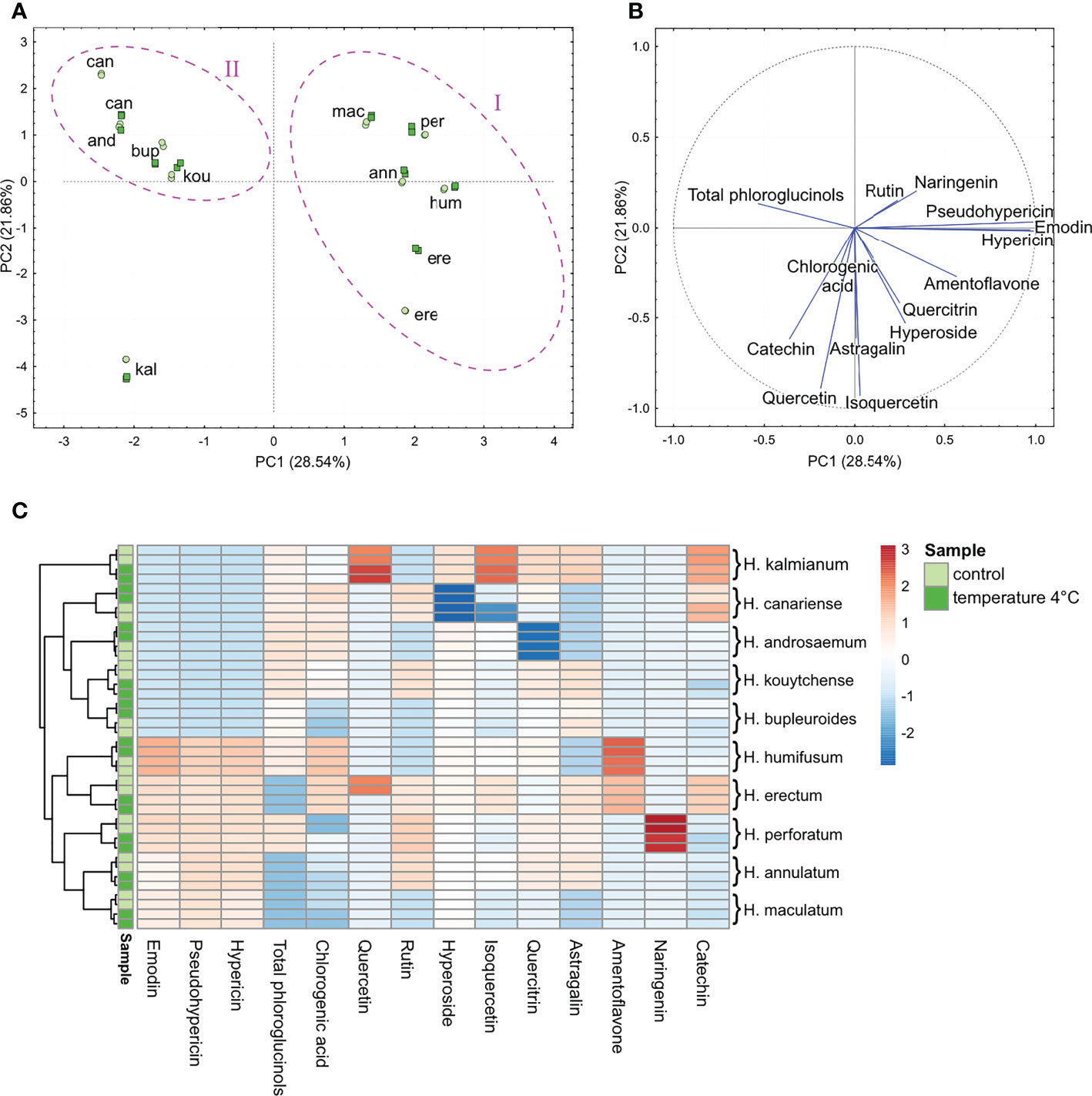
Figure 2 The PCA (A, B) and HCA (C) analyses of anthraquinones, phloroglucinols, chlorogenic acid and flavonoids quantified in the extracts of shoots of in vitro-grown H.ypericum spp. Clustering of species (cases) is highlighted with a magenta ellipse drawn around each cluster (A) in relation to metabolite contents (variables) (B). Each species is represented by two biological replicates (two points per species). The HCA dendrogram (C) shows clustering of the species according to the branches. Each square in the heatmap dendrogram (C) represents the relative content of a metabolite. The red and blue color represent an increase and a decrease of relative metabolite content. Light green and dark green color of the samples indicate the metabolite contents in control plants and plants exposed to temperature of 4°C. (For interpretation of the references to color in this figure legend, the reader is referred to the Web version of this article.) kou, H. kouytchense; and, H. androsaemum; bup, H. bupleuroides; per, H. perforatum; mac, H. maculatum; ere, H. erectum; hum, H. humifusum; kal, H. kalmianum; can, H. canariense; ann, H. annulatum.
According to Figure 2A, more than 50% of the variance was accounted for the first two components PC1 and PC2. The species found in the 2nd and 4th quadrants formed a relatively compact group corresponding to cluster I, representing the sections Hypericum (H. perforatum, H. maculatum, H. erectum), Adenosepalum (H. annulatum) and Oligostema (H. humifusum). The remaining representatives seen in the 1st quadrant formed the separate cluster II comprising the sections Webbia (H. canariense), Androsaemum (H. androsaemum), Bupleuroides (H. bupleuroides) and Ascyreia (H. kouytchense). Based on the relative metabolite content, H. kalmianum (Myriandra) was the most distantly related species, forming a singleton in the 3rd quadrant. The HCA dendrogram confirmed PCA clustering showing two separate clades of hierarchically nested species and H. kalmianum as a separate branch (Figure 2C). Using both the PCA and HCA hierarchical heatmap, the determinant metabolites were identified for each cluster (Figures 2B, C). Naphthodianthrones and their potential precursor emodin were identified as the main determinants for grouping the species in cluster I. The phloroglucinol derivatives were the major profiling compounds of species belonging to cluster II. The flavonoid quercetin, and its glycoside isoquercetin, along with catechin and astragalin (kaempferol-3-O-glucoside), were the most abundant metabolites in H. kalmianum.
As we have shown previously, H. kalmianum, H. perforatum and H. humifusum were characterized as FT species; for example, H. kalmianum plants tolerated ice crystallization up to -11°C (Petijová et al., 2014). In the context of metabolic adaptations related to the freezing tolerance strategy, a relatively higher basal level of the flavonols quercetin and its glycosides, as well as astragalin in H. kalmianum suggests an interconnection between the flavonoids accumulation and plant ability to tolerate ice crystallization. Additionally, the elevated basal content of naringenin and amentoflavone was observed in other FT representatives, e.g., H. perforatum and H. humifusum. On the other side, no substantially elevated basal level of flavonoids was seen in H. canariense or H. kouytchense, which are known as FS species avoiding freezing by supercooling (Petijová et al., 2014).
In Hypericum plants exposed to 4°C, the HCA dendrograms did not reveal any substantial metabolic alterations excepting the enhanced quercetin content in H. kalmianum (Figure 2C). It should be noticed that the subjection of plants to 4°C for 7 days did not represent any lethal stress for Hypericum plants involved in this study. The plants did not change their habitus and continued their growth. However, the exposure of FT Hypericum spp. to temperature of 4°C for several days induced the processes typical for the cold acclimation (Bruňáková et al., 2011; Petijová et al., 2014). Under cold acclimation, a major reprogramming of the transcriptome, proteome, and metabolome results in the accumulation of various cryoprotective substances like saccharides, saccharide alcohols, low-molecular weight nitrogenous compounds, amino acids including proline, cold-regulated (COR) proteins, antioxidant enzymes, polyamines, and polyphenolic metabolites, including several subgroups of flavonoids (Fürtauer et al., 2019; Xu and Fu, 2022).
The relationship between the enhanced accumulation of flavonoids and plant tolerance to the under-zero temperatures is known and well documented. In the model species Arabidopsis thaliana, the flavonols, particularly kaempferols and quercetin glycosides, along with the anthocyanins, and phenylpropanoids were shown to be involved in the freezing tolerance by increasing the antioxidant capacity of tissues exposed to low temperatures (Schulz et al., 2015; Schulz et al., 2016; Zhang et al., 2019; Schulz et al., 2021). Apart from Arabidopsis, higher flavonoid contents, including flavonoid aglycones and glycosides, was associated with freezing tolerance in the number of species, for example Primula malacoides (Isshiki et al., 2014) or Rhododendron cultivars (Swiderski et al., 2004).
Despite the causal relationship between the accumulation of flavonoids and freezing tolerance has not been revealed yet, flavonoids have been successfully applied for prediction of the freezing strategy. For example, in A. thaliana, the unique combinations of these compounds were proposed as metabolic markers of the freezing tolerance (Korn et al., 2008; Schulz et al., 2016). The metabolic interactions between saccharides and plant SMs suggest a possible relationship between accumulation of flavonoid glycosides and enhanced tolerance to freezing in cold-acclimated plants (Korn et al., 2008; Fürtauer et al., 2019). Among the mechanisms, by which plant polyphenols contribute to the protection of plants against a freezing damage, the flavonoids and phenolic acids are directly involved in scavenging free radicals, modulation of the phytohormone-mediated stress responses, stabilization of the cell membranes, or preventing the proteins aggregation (Sharma et al., 2012; Hasanuzzaman et al., 2020).
On the other side, the molecular, biochemical, and physiological changes induced by cold acclimation lead to suppression of some subgroups of SMs accumulation in FT plants exposed to low but above-zero temperatures (Fürtauer et al., 2019). In our previous research, the subjection of FT Hypericum spp. to 4°C resulted in the decrease of some SMs, e.g., carotenoids and naphthodianthrones (Petijová et al., 2014; Bruňáková et al., 2015). Similarly, a decrease of terpene indole alkaloids content was seen in Catharanthus roseus plants under acclimated conditions (Dutta et al., 2013).
3.2 Antioxidant profiling of plants exposed to 4°C
The PCA and HCA revealed a naturally occurring interspecific variability in the basal level of non-enzymatic AOXs (proline and carotenoids), and enzymatic activities (CAT, SOD and APX). As shown by the PCA, more than 60% of the variability could be explained by PC1 and PC2 (Figure 3A). Within the control group of plants without the cold exposure, H. kalmianum formed a clearly separated singleton in the 1st quadrant based on the contribution of the profiling metabolites (Figures 3A, B). The other species were mostly situated in the 2nd quandrant (Figure 3A). According to the HCA dendrogram and HCA-based heatmap, the control plants of H. kalmianum represented a separate branch due to substantially higher basal level of APX activity. The cold-treated plants formed a common and relatively compact cluster spreading among all four quadrants (Figure 3A). The HCA heatmap revealed a substantial elevation of AOX enzymatic activities, along with the increased content of proline and carotenoids, which followed exposure of Hypericum plants to 4°C (Figure 3C).
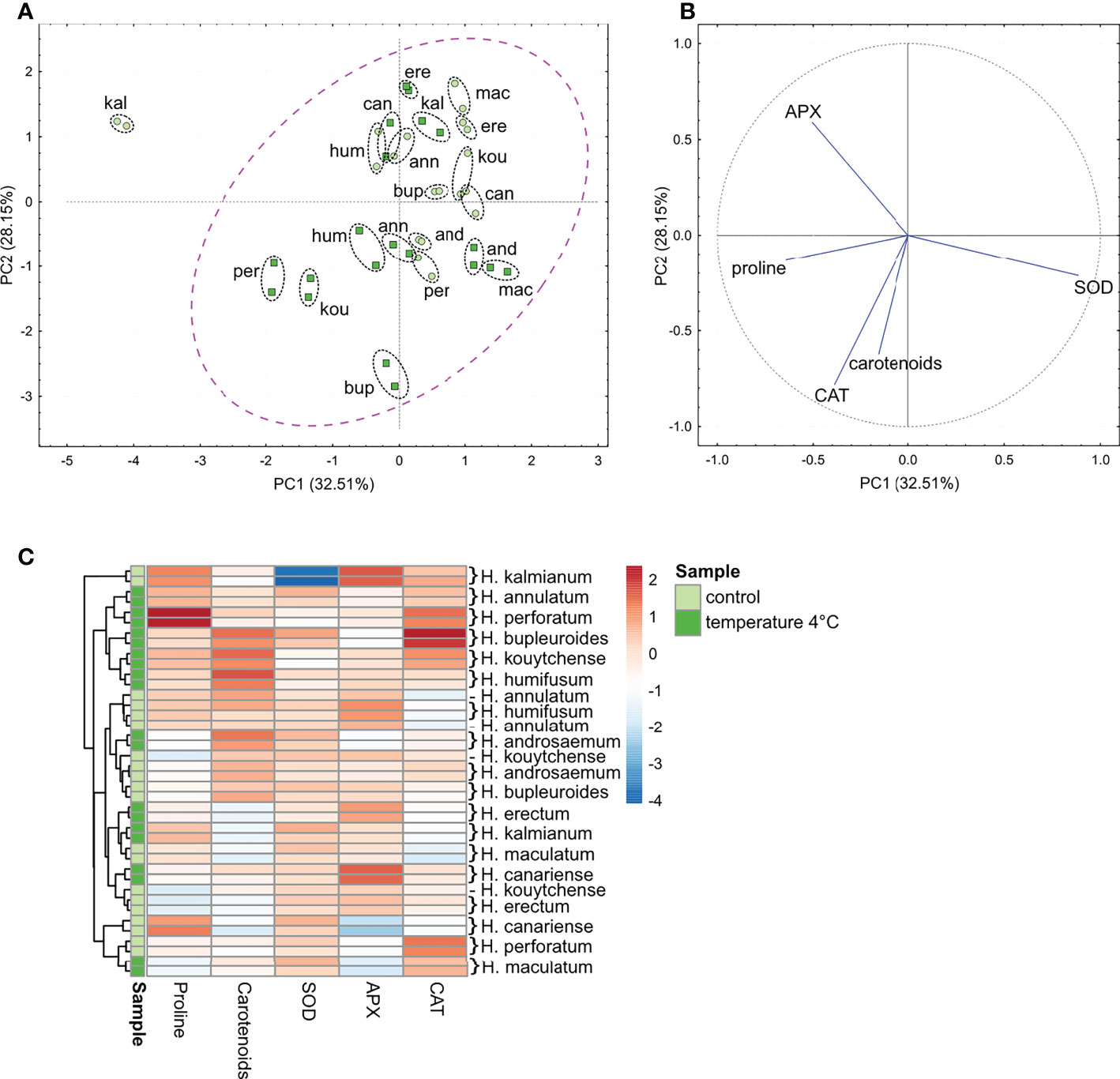
Figure 3 The PCA (A, B) and HCA (C) analyses of non-enzymatic (proline, carotenoids) and enzymatic (SOD, CAT, APX) antioxidant systems assessed in the extracts of shoots of in vitro-grown H.ypericum spp. A magenta-colored ellipse is drawn around the cluster of most of the species (cases) (A) in relation to content of non-enzymatic antioxidants, and activity of antioxidant enzymes (variables) (B). Each species is represented by two biological replicates (highlighted with a black ellipse drawn around each pair of points per species). The HCA dendrogram (C) shows clustering of the species according to the branches. Each square in the heatmap dendrogram (C) represents relative content of a non-enzymatic antioxidants, or activity of antioxidant enzymes; an increase and a decrease is represented by the red and blue color. Light green and dark green color of the samples indicate the variables in control plants and plants exposed to temperature of 4°C. (For interpretation of the references to color in this figure legend, the reader is referred to the Web version of this article.) kou, H. kouytchense; and, H. androsaemum; bup, H. bupleuroides; per, H. perforatum; mac, H. maculatum; ere, H. erectum; hum, H. humifusum; kal, H. kalmianum; can, H. canariense; ann, H. annulatum.
3.2.1 Non-enzymatic AOXs
In relation to the adaptation strategy, the cold exposure led to the highest proline accumulation in FT H. perforatum, while the carotenoids increased in the species exhibiting either the tolerance (H. humifusum) or avoidance defense mechanisms (H. kouytchense, H. androsaemum) (Figure 3C).
The relationship between non-enzymatic AOXs and freezing tolerance has not been fully understood yet. In common, proline is one of the main osmolytes contributing to the enhanced cold tolerance in plants (Verbruggen and Hermans, 2008). However, the content of proline does not indispensably correlate with the extent of freezing tolerance and depends on previous cold exposure of FT plants. The cytosolic proline increased in non-acclimated A. thaliana plants, but no correlation was found between the level of proline and elevated freezing tolerance in cold-acclimated plants (Hoermiller et al., 2022).
On the other hand, the level of carotenoids and ABA were shown to be in positive correlation with enhanced tolerance to cold stress e.g. in Oryza sativa ssp. indica possessing the tolerance strategy (Pan et al., 2022). Carotenoids are pigments, which act in essential physiological processes and plant antioxidant protection during various stresses. Being the important light harvesters, carotenoids have a crucial role in photoprotection of plants; they scavenge the ROS produced by triplet excited chlorophylls, dissipate the excess of absorbed energy during stress condition and stabilize the membranes (Uarrota et al., 2018). Besides, carotenoids are universal precursors of plant hormones including ABA that is known to be involved in hormone signal transduction in plants under cold conditions (Guo et al., 2018). However, a significant decrease of total carotenoids content was observed in FT H. perforatum or FS H. canariense plants exposed to the cold acclimation regime in our previous research (Bruňáková et al., 2015). In plants, the level of photosynthetic pigments depends on many factors; even in tolerant plants, the content of chlorophylls and carotenoids could be reduced in relation to the temperature decrease and duration of cold stress (Lukatkin et al., 2012). As an example, the temperature decline from 0°C to −4°C led to a partial reduction in the content of leaf carotenoids in Vicia faba tolerating freezing stress up to −10°C (Nabati et al., 2021). In sensitive plants, the cold stress usually leads to photosynthetic disruption (Wise and Naylor, 1987).
3.2.2 Enzymatic AOXs
In Hypericum spp., we observed a substantial variability in the APX, CAT and SOD activities. Among the control plants, the highest basal activity of APX and CAT were seen in FT species H. kalmianum and H. perforatum, respectively (Figure 3C). The exposure of plants to a temperature of 4°C for 7 days led to various changes in antioxidant enzymatic activities in Hypericum representatives differing in the freezing adaptation strategies. The activity of SOD significantly elevated in H. kalmianum exhibiting strong freezing tolerance. The APX increased in H. canariense using avoidance strategy, and the CAT markedly rised in H. bupleuroides with the unknown mechanism of preventing the freezing injury.
Without the requirement of a cold acclimation, the naturally higher levels of enzymatic antioxidants might reflect the genetically predetermined stress response of plants under unstable environmental conditions. For example, the thylakoid membrane bound APXs (tAPXs) represent the extra-plastidic stress protection against a sudden decrease of temperature (van Buer et al., 2016). Coincidently, Chen et al. (2014) documented higher CAT activity in non-acclimated plants of Chrysanthemum dichrum, the species exhibiting a strong frost tolerance when compared with the weak frost resistant Chrysanthemum makinoi.
When the ambient temperature declines towards 0°C, plants use different enzymatic antioxidant systems to scavenge excessive ROS to minimize the oxidative stress in tissues (Xu et al., 2015; Karim and Johnson, 2021). Upon cold acclimation, the elevation of antioxidant enzymatic activities is well documented in the number of species that tolerate freezing, for example Haberlea rhodopensis (Georgieva et al., 2021), Chrysanthemum spp. (Chen et al., 2014), or Eucalyptus spp. (Oberschelp et al., 2020). Along with accumulation of osmolytes, the antioxidant enzymatic activities involving SOD, APX, and CAT, represent the main processes involved in plant tolerance to environmental stresses (Ahmad et al., 2010; Khaleghi et al., 2019).
The functional role of enzymatic AOXs in abiotic stress response of several Hypericum species has been well documented (Danova et al., 2012; Skyba et al., 2012; Georgieva et al., 2014). In H. perforatum tolerant to cryogenic treatment, a considerable intraspecific variability was seen related to the SOD, CAT, malondialdehyde (MDA), total free proline and carotenoids, suggesting the genotype-dependent differences in physiological and biochemical adaptations to the low-temperature treatment (Skyba et al., 2010).
In front-line of defense against ROS induced by cold stress, the SOD metalloenzymes represent the key enzymes controlling the oxidative status in higher plants (Sen Raychaudhuri and Deng, 2000). In plant cells, the SODs comprising the manganese (Mn-SOD), iron (Fe-SOD) and copper/zinc (Cu/Zn-SOD) catalyze the dismutation of O2•- to less reactive products like O2 and H2O2 (Bueno et al., 1995; Alscher et al., 2002). The functional role of SOD in adaptation to cold stress conditions was reported for model plants like Lycopersicum esculentum (Soydam Aydin et al., 2013), Nicotiana tabacum (Wei et al., 2022), A. thaliana (Gill et al., 2010), or other plant species, for example a Himalayan high altitude alpine plant Potentilla atrosanguinea variety argyrophylla (Sahoo et al., 2001). According to Berwal and Ram (2018), the total SOD activity can be applied as an important biochemical marker indicating abiotic stress tolerance in higher plants. Moreover, the SOD isoenzyme profiles are commonly used as the selection criterion for screening plants for higher level of tolerance to various abiotic stimuli, including heat, cold, drought, salinity and heavy metal contaminants (Saed-Moucheshi et al., 2021).
The enzymatic defense including APX, and CAT contribute to an increased capacity of plant antioxidant tolerance to various environmental stresses (Yang et al., 2009; Yin et al., 2010). The APX and CAT are principal plant antioxidant H2O2-scavenging enzymes differing in their afinity to H2O2 and other organic peroxides (Sharma et al., 2012). In FT species H. perforatum and H. kalmianum, no increase in either CAT or APX activities was detected after exposure to the cold acclimation temperature of 4°C indicating that the CATs and APXs might represent an integral part of antioxidant defense in Hypericum species that acclimate after exposure to low but non-freezing temperatures (Figure 3C). It has been shown that the plant AOXs, such as the CATs normally operate below their maximum capacity, even if H2O2 concentrations reach relatively high values (Mhamdi et al., 2010). On the other side, when FS Hypericum plants were subjected to 4°C, higher CAT and APX activities were observed in H. canariense and H. kouytchense, respectively. An increase in CAT, APX or other antioxidant enzymatic activities might signalize the oxidative burst in plant tissues indicating the mechanism by which sensitive plants compensate higher level of ROS during cold exposure. For example, the H2O2 and MDA content increased more in the sensitive genotypes of Hordeum vulgare than in the tolerant ones (Valizadeh et al., 2017). However, the enhancement or depletion of H2O2-scavenging enzymes depends on several factors including the type, intensity, and duration of stress stimuli, which should be taken into consideration (Bayat et al., 2018).
3.3 Prediction of tolerance to cold stress – concluding remarks
In response to acclimation temperature of 4°C, the metabolic and antioxidant phenotyping revealed substantial alterations in basal level of phenolic compounds, along with other AOXs non-enzymatic components and enzymatic activities among Hypericum species differing in their geographical distribution. In relation to phenolic composition, the flavonols quercetin and quercetin glycosides, as well as astragalin, and other flavonoid metabolites like naringenin and amentoflavone could be considered as metabolites contributing to the freezing tolerance in the genus Hypericum. Higher basal level of proline, carotenoids and enzymatic activities of SOD, CAT and APX might reflect the genetically predetermined mechanisms of a stress response in tolerant species or signalize the oxidative burst in sensitive Hypericum representatives.
The tolerance to cold stress is a highly complex trait influenced by multiple factors; the reconfiguration of primary and secondary metabolic pathways along with activation of antioxidant enzymatic defense usually reflect the genetically predetermined capacity of the species adaptation to the local stress conditions. Based on electrolyte leakage (LT50 values), the freezing adaptation strategy was shown to be consistent with the natural habitat of a species. Within the genus Hypericum, the extent of the freezing tolerance was shown to concur with the post-cryogenic regeneration reflecting the geographical distribution of a species (Petijová et al., 2014; Bruňáková et al., 2015). While the (sub)tropical H. canariense, endemic of Canary Island and Madeira (Robson, 1996), was referred as cold-sensitive species avoiding ice formation by supercooling, other studied Hypericum species originating from the temperate zones, or growing at higher altitudes of the subtropical areas were shown to be relatively tolerant to freezing (Petijová et al., 2014). For example, the nearly cosmopolitan H. perforatum, and H. kalmianum restricted to cold area of USA and Canada (adjacent to Ontario Lake and Ottawa River) were characterized as the FT species (Petijová et al., 2014; Bruňáková et al., 2015). However, the prediction of freezing resistance strategy based on metabolic and antioxidant profiling is usually complicated by a large intraspecific variability related to the origin of the plant accession. The conflicting evidence of tolerance/avoidance mechanism was reported for A. thaliana accessions originated from different habitats (Zhen and Ungerer, 2008; Hoermiller et al., 2018). As shown by Nägele and Heyer (2013), the cold-induced intensive accumulation of saccharides and amino acids was higher in FT accessions of A. thaliana when compared with their FS counterparts. Significant alterations in AOX metabolism were also observed between FT and FS cultivars of Hordeum vulgare (Dai et al., 2009).
In conclusion, we assume that the combination of metabolic and enzymatic AOXs, along with other physiological markers including the thermal properties of plant tissues (LT50), and knowledge of the plant origin could be used for prediction of a mechanism, by which Hypericum spp. adapt to a cold stress. Albeit some classes of phenolic compounds contribute to the enhanced stress tolerance, more data are needed to identify the unique combinations of metabolites that would indirectly allow to estimate the extent of freezing tolerance of a particular species in the genus Hypericum.
4 Summary
Even though the genus Hypericum belongs to most diverse plant taxa, rare and endemic representatives might be threatened due to increasing climate changes contributing to the destruction of their natural habitats. In common, plant adaptation to changing environment depends on the activation of the cascades of molecular networks related to stress perception, signal transduction, induction of enzymatic activity and biosynthesis of SMs involved in the acquired systemic plant responses. Although the knowledge of AOX systems responsive to a particular stressor, such as low temperature, is critical, the assessment of a combination of several metabolic, physiological, and molecular markers is needed to understand the molecular mechanisms of the overall biotic/abiotic stress tolerance. The metabolic and antioxidant profiling of Hypericum spp. revealed a great species-dependent and environmentally influenced variability that usually complicates the application of phenotyping to estimate the plant stress tolerance. Therefore, the stress-induced changes in metabolic and antioxidant profiles should always be evaluated in a complex manner; in addition to the species-specific structural or physiological predeterminations, the applicability of profiling metabolites is also crucial. Within the genus, the most prominent stress-induced responses are usually associated with the accumulation of polyphenolic compounds including the chlorogenic acid, and flavonoids, namely the amentoflavone, quercetin or kaempferol glycosides, indicating their function in tolerance against various biotic/abiotic stresses. Although current mathematical models revealed contribution of genetic and environmental factors, more data are needed for relevant prediction of biosynthetic capacity of Hypericum spp. in relation to overall stress tolerance.
Author contributions
KB summarized up-to-date knowledge on the topic, MB performed all the experiments and HPLC analysis of plant material. MB, KB, and LP statistically analysed and interpreted chemical data. MB, KB and LP prepared the figures and tables. EČ conceived the project and coordinated all stages of the experimental work. MB and KB drafted the manuscript and EČ revised and approved the manuscript. All authors contributed to the article and approved the submitted version.
Funding
This work was supported by the Scientific Grant Agency (VEGA) of the Ministry of Education of Slovakia [grant number 1/0013/19] and the Slovak Research and Development Agency (APVV) [grant number APVV-18-0125].
Acknowledgments
The authors wish to thankfully acknowledge RNDr. Viktória Bul'ková for her assistance with chemical analyses of the samples.
Conflict of interest
The authors declare that the research was conducted in the absence of any commercial or financial relationships that could be construed as a potential conflict of interest.
Publisher’s note
All claims expressed in this article are solely those of the authors and do not necessarily represent those of their affiliated organizations, or those of the publisher, the editors and the reviewers. Any product that may be evaluated in this article, or claim that may be made by its manufacturer, is not guaranteed or endorsed by the publisher.
Supplementary material
The Supplementary Material for this article can be found online at: https://www.frontiersin.org/articles/10.3389/fpls.2022.1042375/full#supplementary-material
References
Agapouda, A., Booker, A., Kiss, T., Hohmann, J., Heinrich, M., Csupor, D. (2019). Quality control of Hypericum perforatum L. analytical challenges and recent progress. J. Pharm. Pharmacol. 71, 15–37. doi: 10.1111/jphp.12711
Ahmad, P., Jaleel, C. A., Salem, M. A., Nabi, G., Sharma, S. (2010). Roles of enzymatic and non-enzymatic antioxidants in plants during abiotic stress. Crit. Rev. Biotechnol. 30, 161–175. doi: 10.3109/07388550903524243
Alscher, R. G., Erturk, N., Heath, L. S. (2002). Role of superoxide dismutases (SODs) in controlling oxidative stress in plants. J. Exp. Bot. 53, 1331–1341. doi: 10.1093/jexbot/53.372.1331
Angelova, Z., Georgiev, S., Roos, W. (2006). Elicitation of plants. Biotechnol. Biotec. Eq. 20, 72–83. doi: 10.1080/13102818.2006.10817345
Ashraf, M., Riaz, M., Arif, M., Rasheed, R., Iqbal, M., Hussain, I., et al. (2019). “The role of non-enzymatic antioxidants in improving abiotic stress tolerance in plants,” in Plant tolerance to environmental stress. Eds. Hasanuzzaman, M., Fujita, M., Oku, H., Islam, T. M. (Boca Raton: CRC Press). doi: 10.1201/9780203705315-9
Azeez, H. A., Ibrahim, K. M. (2013). Effect of biotic elicitors on secondary metabolite production in cell suspensions of Hypericum triquetrifolium Turra. Bull. Univ. Agric. Sci. Vet. Med. Cluj-Napoca Hortic. 70, 26–33. doi: 10.15835/BUASVMCN-HORT:9264
Bálintová, M., Bruňáková, K., Petijová, L., Čellárová, E. (2019). Targeted metabolomic profiling reveals interspecific variation in the genus Hypericum in response to biotic elicitors. Plant Physiol. Bioch. 135, 348–358. doi: 10.1016/j.plaphy.2018.12.024
Bayat, L., Arab, M., Aliniaeifard, S., Seif, M., Lastochkina, O., Li, T. (2018). Effects of growth under different light spectra on the subsequent high light tolerance in rose plants. AoB PLANTS 10, ply052. doi: 10.1093/aobpla/ply052
Berwal, M. K., Ram, C. (2018). “Superoxide dismutase: a stable biochemical marker for abiotic stress tolerance in higher plants,” in Abiotic and biotic stress in plants. Ed. de Oliveira, A. B. (London: IntechOpen). doi: 10.5772/intechopen.82079
Brechner, M. L., Albright, L. D., Weston, L. A. (2011). Effects of UV-B on secondary metabolites of St. John’s wort (Hypericum perforatum L.) grown in controlled environments. Photochem. Photobiol. 87, 680–684. doi: 10.1111/j.1751-1097.2011.00904.x
Bridi, H., Meirelles, G. C., von Poser, G. L. (2018). Structural diversity and biological activities of phloroglucinol derivatives from Hypericum species. Phytochemistry 155, 203–232. doi: 10.1016/j.phytochem.2018.08.002
Briskin, D. P., Gawienowski, M. C. (2001). Differential effects of light and nitrogen on production of hypericins and leaf glands in Hypericum perforatum. Plant Physiol. Bioch. 39, 1075–1081. doi: 10.1016/S0981-9428(01)01326-2
Bruňáková, K., Čellárová, E. (2016). “Shoot tip meristem cryopreservation of Hypericum species,” in Protocols for in vitro cultures and secondary metabolite analysis of aromatic and medicinal plants: Methods in molecular biology. Ed. Jain, S. M. (New York: Humana Press). doi: 10.1007/978-1-4939-3332-7_3
Bruňáková, K., Čellárová, E. (2017). Modulation of anthraquinones and phloroglucinols biosynthesis in Hypericum spp. by cryogenic treatment. J. Biotechnol. 251, 59–67. doi: 10.1016/j.jbiotec.2017.04.012
Bruňáková, K., Petijová, L., Zámečník, J., Turečková, V., Čellárová, E. (2015). The role of ABA in the freezing injury avoidance in two Hypericum species differing in frost tolerance and potential to synthesise hypericins. Plant Cell Tiss. Org. 122, 45–56. doi: 10.1007/s11240-015-0748-9
Bruňáková, K., Zámečník, J., Urbanová, M., Čellárová, E. (2011). Dehydration status of ABA-treated and cold-acclimated Hypericum perforatum L. shoot tips subjected to cryopreservation. Thermochim. Acta 525, 62–70. doi: 10.1016/j.tca.2011.07.022
Brunetti, C., Di Ferdinando, M., Fini, A., Pollastri, S., Tattini, M. (2013). Flavonoids as antioxidants and developmental regulators: relative significance in plants and humans. Int. J. Mol. Sci. 14, 3540–3555. doi: 10.3390/ijms14023540
Bueno, P., Varela, J., Gimenez-Gallego, G., Del Rio, L. A. (1995). Peroxisomal copper, zinc superoxide dismutase. Characterization of the isoenzyme from watermelon cotyledons. Plant Physiol. 108, 1151–1160. doi: 10.1104/pp.108.3.1151
Bul'ková, V. (2018). Physiological and biochemical status of cold-elicited in vitro systems in the genus Hypericum (Košice: Pavol Jozef Šafárik University).
Carrubba, A., Lazzara, S., Giovino, A., Ruberto, G., Napoli, E. (2021). Content variability of bioactive secondary metabolites in Hypericum perforatum L. Phytochem. Lett. 46, 71–78. doi: 10.1016/j.phytol.2021.09.011
Caverzan, A., Casassola, A., Brammer, S. P. (2016). “Reactive oxygen species and antioxidant enzymes involved in plant tolerance to stress,” in Abiotic and biotic stress in plants. Eds. Shanker, A. K., Shanker, C. (London: IntechOpen). doi: 10.5772/61368
Chandran, A. K. N., Kim, J. W., Yoo, Y. H., Park, H. L., Kim, Y. J., Cho, M. H., et al. (2019). Transcriptome analysis of rice-seedling roots under soil–salt stress using RNA-seq method. Plant Biotechnol. Rep. 13, 567–578. doi: 10.1007/s11816-019-00550-3
Chen, Y., Jiang, J., Chang, Q., Gu, C., Song, A., Chen, S., et al. (2014). Cold acclimation induces freezing tolerance via antioxidative enzymes, proline metabolism and gene expression changes in two chrysanthemum species. Mol. Biol. Rep. 41, 815–822. doi: 10.1007/s11033-013-2921-8
Chung, M. H., Deng, T. S. (2020). Effects of circadian clock and light on melatonin concentration in Hypericum perforatum L. (St. John’s wort). Bot. Stud. 61, 23. doi: 10.1186/s40529-020-00301-6
Ciccarelli, D., Andreucci, A., Pagni, A. (2001). Translucent glands and secretory canals in Hypericum perforatum L. (Hypericaceae): Morphological, anatomical and histochemical studies during the course of ontogenesis. Ann. Bot. - London 88, 637–644. doi: 10.1006/anbo.2001.1514
Çirak, C., Aksoy, H. M., Ayan, A. K., Sağlam, B., Kevseroğlu, K. (2005). Enhanced hypericin production in Hypericum perforatum and Hypericum pruinatum in response to inoculation with two fungal pathogens. Plant Protect. Sci. 41, 109–114. doi: 10.17221/2725-PPS
Çirak, C., Radusiene, J. (2019). Factors affecting the variation of bioactive compounds in Hypericum species. Biol. Fut. 70, 198–209. doi: 10.1556/019.70.2019.25
Çirak, C., Radusiene, J., Arslan, B. (2008). Variation of bioactive substances in Hypericum montbretii during plant growth. Nat. Prod. Res. 22, 246–252. doi: 10.1080/14786410701642623
Çirak, C., Radusiene, J., Ivanauskas, L., Jakstas, V., Camaş, N. (2015). Population variability of main secondary metabolites in Hypericum lydium Boiss. (Hypericaceae). Iran. J. Pharm. Res. 14, 969–978.
Çirak, C., Radusiene, J., Ivanauskas, L., Jakstas, V., Camas, N. (2014). Phenological changes in the chemical content of wild and greenhouse-grown Hypericum pruinatum: Flavonoids. Turk. J. Agric. For. 38, 362–370. doi: 10.3906/tar-1308-17
Çirak, C., Radusiene, J., Jakstas, V., Ivanauskas, L., Seyis, F., Yayla, F. (2016). Secondary metabolites of seven Hypericum species growing in Turkey. Pharm. Biol. 54, 2244–2253. doi: 10.3109/13880209.2016.1152277
Coste, A., Pop, C., Halmagyi, A., Butiuc-Keul, A. (2021). “Secondary metabolites in shoot cultures of Hypericum,” in Plant cell and tissue differentiation and secondary metabolites. Reference series in phytochemistry. Eds. Ramawat, K. G., Ekiert, H. M., Goyal, S. (Cham: Springer). doi: 10.1007/978-3-030-30185-9_9
Coste, A., Vlase, L., Halmagyi, A., Deliu, C., Coldea, G. (2011). Effects of plant growth regulators and elicitors on production of secondary metabolites in shoot cultures of Hypericum hirsutum and Hypericum maculatum. Plant Cell Tiss. Org. 106, 279–288. doi: 10.1007/s11240-011-9919-5
Couceiro, M. A., Afreen, F., Zobayed, S. M. A., Kozai, T. (2006). Variation in concentrations of major bioactive compounds of St. John’s wort: effects of harvesting time, temperature and germplasm. Plant Sci. 170, 128–134. doi: 10.1016/j.plantsci.2005.08.011
Crockett, S. L., Robson, N. K. (2011). Taxonomy and chemotaxonomy of the genus Hypericum. Med. Aromat. Plant Sci. Biotechnol. 5, 1–13.
Cui, X. H., Murthy, H. N., Wu, C. H., Paek, K. Y. (2010). Sucrose-induced osmotic stress affects biomass, metabolite, and antioxidant levels in root suspension cultures of Hypericum perforatum L. Plant Cell Tiss. Org. 103, 7–14. doi: 10.1007/s11240-010-9747-z
Dai, F., Huang, Y., Zhou, M., Zhang, G. (2009). The influence of cold acclimation on antioxidative enzymes and antioxidants in sensitive and tolerant barley cultivars. Biol. Plant 53, 257–262. doi: 10.1007/s10535-009-0048-5
Danova, K., Nikolova-Damianova, B., Denev, R., Dimitrov, D. (2012). Influence of vitamins on polyphenolic content, morphological development, and stress response in shoot cultures of Hypericum spp. Plant Cell Tiss. Org. 110, 383–393. doi: 10.1007/s11240-012-0159-0
de Abreu, I. N., Mazzafera, P. (2005). Effect of water and temperature stress on the content of active constituents of Hypericum brasiliense Choisy. Plant Physiol. Bioch. 43, 241–248. doi: 10.1016/j.plaphy.2005.01.020
de Abreu, I. N., Porto, A. L. M., Marsaioli, A. J., Mazzafera, P. (2004). Distribution of bioactive substances from Hypericum brasiliense during plant growth. Plant Sci. 167, 949–954. doi: 10.1016/j.plantsci.2004.06.002
DeLuna, S. L., Ramírez-Garza, R. E., Saldívar, S. O. S. (2020). Environmentally friendly methods for flavonoid extraction from plant material: Impact of their operating conditions on yield and antioxidant properties. Sci. World J. 2020, 6792069. doi: 10.1155/2020/6792069
Ding, Y., Shi, Y., Yang, S. (2019). Advances and challenges in uncovering cold tolerance regulatory mechanisms in plants. New Phytol. 222, 1690–1704. doi: 10.1111/nph.15696
Dussarrat, T., Prigent, S., Latorre, C., Bernillon, S., Flandin, A., Díaz, F. P., et al. (2022). Predictive metabolomics of multiple Atacama plant species unveils a core set of generic metabolites for extreme climate resilience. New Phytol. 234, 1614–1628. doi: 10.1111/nph.18095
Dutta, A., Sen, J., Deswal, R. (2013). New evidences about strictosidine synthase (Str) regulation by salinity, cold stress and nitric oxide in Catharanthus roseus. J. Plant Biochem. Biot. 22, 124–131. doi: 10.1007/s13562-012-0118-1
Fang, X., Yang, C. Q., Wei, Y. K., Ma, Q. X., Yang, L., Chen, X. Y. (2011). Genomics grand for diversified plant secondary metabolites. Plant Div. Res. 33, 53–64. doi: 10.3724/SP.J.1143.2011.10233
Farnese, F. S., Menezes-Silva, P. E., Gusman, G. S., Oliveira, J. A. (2016). When bad guys become good ones: The key role of reactive oxygen species and nitric oxide in the plant responses to abiotic stress. Front. Plant Sci. 7. doi: 10.3389/fpls.2016.00471
Ferdinando, M. D., Brunetti, C., Fini, A., Tattini, M. (2012). “Flavonoids as antioxidants in plants under abiotic stresses,” in Abiotic stress responses in plants: Metabolism, productivity and sustainability. Eds. Ahmad, P., Prasad, M. N. V. (New York, NY: Springer). doi: 10.1007/978-1-4614-0634-1_9
Fernandez, O., Urrutia, M., Bernillon, S., Giauffret, C., Tardieu, F., Le Gouis, J., et al. (2016). Fortune telling: Metabolic markers of plant performance. Metabolomics 12, 158. doi: 10.1007/s11306-016-1099-1
Franklin, G., Conceição, L. F. R., Kombrink, E., Dias, A. C. P. (2009). Xanthone biosynthesis in Hypericum perforatum cells provides antioxidant and antimicrobial protection upon biotic stress. Phytochemistry 70, 60–68. doi: 10.1016/j.phytochem.2008.10.016
Fürtauer, L., Weiszmann, J., Weckwerth, W., Nägele, T. (2019). Dynamics of plant metabolism during cold acclimation. Int. J. Mol. Sci. 20, 5411. doi: 10.3390/ijms20215411
Gadzovska, S., Maury, S., Delaunay, A., Spasenoski, M., Hagege, D., Courtois, D., et al. (2013). The influence of salicylic acid elicitation of shoots, callus, and cell suspension cultures on production of naphtodianthrones and phenylpropanoids in Hypericum perforatum L. Plant Cell Tiss. Org. 113, 25–29. doi: 10.1007/s11240-012-0248-0
Gadzovska, S., Maury, S., Delaunay, A., Spasenoski, M., Joseph, C., Hagege, D. (2007). Jasmonic acid elicitation of Hypericum perforatum L. cell suspensions and effect on the production of phenylpropanoids and naphtodianthrones. Plant Cell Tiss. Org. 89, 1–13. doi: 10.1007/s11240-007-9203-x
Gadzovska-Simic, S., Tusevski, O., Antevski, S., Atanasova-Pancevska, N., Petreska, J., Stefova, M., et al. (2012). Secondary metabolite production in Hypericum perforatum L. cell suspensions upon elicitation with fungal mycelia from Aspergillus flavus. Arch. Biol. Sci. 64, 113–121. doi: 10.2298/ABS1201113G
Gadzovska-Simic, S., Tusevski, O., Maury, S., Delaunay, A., Joseph, C., Hagège, D. (2014). Effects of polysaccharide elicitors on secondary metabolite production and antioxidant response in Hypericum perforatum L. shoot cultures Sci. World J. 2014, 609649. doi: 10.1155/2014/609649
Gadzovska-Simic, S., Tusevski, O., Maury, S., Delaunay, A., Lainé, E., Joseph, C., et al. (2015a). Polysaccharide elicitors enhance phenylpropanoid and naphtodianthrone production in cell suspension cultures of Hypericum perforatum. Plant Cell Tiss. Org. 122, 649–663. doi: 10.1007/s11240-015-0798-z
Gadzovska-Simic, S., Tusevski, O., Maury, S., Hano, C., Delaunay, A., Chabbert, B., et al. (2015b). Fungal elicitor-mediated enhancement in phenylpropanoid and naphtodianthrone contents of Hypericum perforatum L. cell cultures. Plant Cell Tiss. Org. 122, 213–226. doi: 10.1007/s11240-015-0762-y
Gago, J., Fernie, A. R., Nikoloski, Z., Tohge, T., Martorell, S., Escalona, J. M., et al. (2017). Integrative field scale phenotyping for investigating metabolic components of water stress within a vineyard. Plant Methods 13, 90. doi: 10.1186/s13007-017-0241-z
Galano, A., Reiter, R. J. (2018). Melatonin and its metabolites vs oxidative stress: From individual actions to collective protection. J. Pin. Res. 65, e12514. doi: 10.1111/jpi.12514
Gao, J., Liu, Z. L., Zhao, W., Tomlinson, K. W., Xia, S. W., Zeng, Q. Y., et al. (2021). Combined genotype and phenotype analyses reveal patterns of genomic adaptation to local environments in the subtropical oak Quercus acutissima. J. Syst. Evol. 59, 541–556. doi: 10.1111/jse.12568
Georgieva, K., Mihailova, G., Gigova, L., Dagnon, S., Simova-Stoilova, L., Velitchkova, M. (2021). The role of antioxidant defense in freezing tolerance of resurrection plant Haberlea rhodopensis. Physiol. Mol. Biol. Pla. 27, 1119–1133. doi: 10.1007/s12298-021-00998-0
Georgieva, E., Petrova, D., Yordanova, Z., Kapchina-Toteva, V., Čellárová, E., Chaneva, G. (2014). Influence of cryopreservation on the antioxidative activity of in vitro cultivated Hypericum species. Biotechnol. Biotec. Eq. 28, 863–870. doi: 10.1080/13102818.2014.946805
Germ, M., Stibilj, V., Kreft, S., Gaberščik, A., Kreft, I. (2010). Flavonoid, tannin and hypericin concentrations in the leaves of St. John’s wort (Hypericum perforatum L.) are affected by UV-B radiation levels. Food Chem. 122, 471–474. doi: 10.1016/j.foodchem.2010.03.008
Gill, T., Sreenivasulu, Y., Kumar, S., Ahuja, P. S. (2010). Over-expression of superoxide dismutase exhibits lignifications of vascular structures in Arabidopsis thaliana. J. Plant Physiol. 167, 757–760. doi: 10.1016/j.jplph.2009.12.004
Gray, D. E., Pallardy, S. G., Garrett, H. E., Rottinghaus, G. E. (2003). Effect of acute drought stress and time of harvest on phytochemistry and dry weight of St. John’s wort leaves and flowers. Planta Med. 69, 1024–1030. doi: 10.1055/s-2003-45150
Guo, X., Liu, D., Chong, K. (2018). Cold signaling in plants: Insights into mechanisms and regulation. J. Integr. Plant Biol. 60, 745–756. doi: 10.1111/jipb.12706
Gusta, L. V., Wisniewski, M. (2013). Understanding plant cold hardiness: An opinion. Physiol. Plantarum. 147, 4–14. doi: 10.1111/j.1399-3054.2012.01611.x
Hall, R. D., D'Auria, J. C., Silva Ferreira, A. C., Gibon, Y., Kruszka, D., Mishra, P., et al. (2022). High-throughput plant phenotyping: A role for metabolomics? Trends Plant Sci. 27, 549–563. doi: 10.1016/j.tplants.2022.02.001
Han, Q. H., Huang, B., Ding, C. B., Zhang, Z. W., Chen, Y. E., Hu, C., et al. (2017). Effects of melatonin on anti-oxidative systems and photosystem II in cold-stressed rice seedlings. Front. Plant Sci. 8. doi: 10.3389/fpls.2017.00785
Hasanuzzaman, M., Bhuyan, M., Zulfiqar, F., Raza, A., Mohsin, S. M., Mahmud, J. A., et al. (2020). Reactive oxygen species and antioxidant defense in plants under abiotic stress: Revisiting the crucial role of a universal defense regulator. Antioxidants (Basel). 9, 681. doi: 10.3390/antiox9080681
Henzelyová, J., Čellárová, E. (2018). Modulation of naphthodianthrone biosynthesis in hairy root-derived Hypericum tomentosum regenerants. Acta Physiol. Plant 40, 82. doi: 10.1007/s11738-018-2664-1
Hoermiller, I. I., Funck, D., Schönewolf, L., May, H., Heyer, A. G. (2022). Cytosolic proline is required for basal freezing tolerance in Arabidopsis. Plant Cell Environ. 45, 147–155. doi: 10.1111/pce.14196
Hoermiller, I. I., Ruschhaupt, M., Heyer, A. G. (2018). Mechanisms of frost resistance in Arabidopsis thaliana. Planta 248, 827–835. doi: 10.1007/s00425-018-2939-1
Isah, T. (2019). Stress and defense responses in plant secondary metabolites production. Biol. Res. 52, 39. doi: 10.1186/s40659-019-0246-3
Isshiki, R., Galis, I., Tanakamaru, S. (2014). Farinose flavonoids are associated with high freezing tolerance in fairy primrose (Primula malacoides) plants. J. Integr. Plant Biol. 56, 181–188. doi: 10.1111/jipb.12145
Jafarirad, S., Kosari-Nasab, M., Mohammadpour Tavana, R., Mahjouri, S., Ebadollahi, R. (2021). Impacts of manganese bio-based nanocomposites on phytochemical classification, growth and physiological responses of Hypericum perforatum L. shoot cultures. Ecotox. Environ. Safe 209, 111841. doi: 10.1016/j.ecoenv.2020.111841
Jan, R., Asaf, S., Numan, M., Lubna, L., Kim, K.-M. (2021a). Plant secondary metabolite biosynthesis and transcriptional regulation in response to biotic and abiotic stress conditions. Agronomy 11, 968. doi: 10.3390/agronomy11050968
Jan, R., Kim, N., Lee, S. H., Khan, M. A., Asaf, S., Lubna, L., et al. (2021b). Enhanced flavonoid accumulation reduces combined salt and heat stress through regulation of transcriptional and hormonal mechanisms. Front. Plant Sci. 12. doi: 10.3389/fpls.2021.796956
Karakas, O., Toker, Z., Tilkat, E., Ozen, H. C., Onay, A. (2009). Effects of different concentrations of benzylaminopurine on shoot regeneration and hypericin content in Hypericum triquetrifolium Turra. Nat. Prod. Res. 23, 1459–1465. doi: 10.1080/14786410701664528
Karim, M. F., Johnson, G. N. (2021). Acclimation of photosynthesis to changes in the environment results in decrease of oxidative stress in Arabidopsis thaliana. Front. Plant Sci. 12. doi: 10.3389/fpls.2021.683986
Kerchev, P. I., Van Breusegem, F. (2022). Improving oxidative stress resilience in plants. Plant J. 109, 359–372. doi: 10.1111/tpj.15493
Khaleghi, A., Naderi, R., Brunetti, C., Maserti, B. E., Salami, S. A., Babalar, M. (2019). Morphological, physiochemical and antioxidant responses of Maclura pomifera to drought stress. Sci. Rep. 9, 19250. doi: 10.1038/s41598-019-55889-y
Khan, A., Numan, M., Khan, A. L., Lee, I. J., Imran, M., Asaf, S., et al. (2020). Melatonin: Awakening the defense mechanisms during plant oxidative stress. Plants 9, 407. doi: 10.3390/plants9040407
Kimáková, K., Kimáková, A., Idkowiak, J., Stobiecki, M., Rodziewicz, P., Marczak, Ł., et al. (2018). Phenotyping the genus Hypericum by secondary metabolite profiling: emodin vs. skyrin, two possible key intermediates in hypericin biosynthesis. Anal. Bioanal. Chem. 410, 7689–7699. doi: 10.1007/s00216-018-1384-0
Kirakosyan, A., Hayashi, H., Inoue, K., Charchoglyan, A., Vardapetyan, H. (2000). Stimulation of the production of hypericins by mannan in Hypericum perforatum shoot cultures. Phytochemistry 53, 345–348. doi: 10.1016/s0031-9422(99)00496-3
Kirakosyan, A., Kaufman, P., Warber, S., Zick, S., Aaronson, K., Bolling, S., et al. (2004). Applied environmental stresses to enhance the levels of polyphenolics in leaves of hawthorn plants. Physiol. Plantarum. 121, 182–186. doi: 10.1111/j.1399-3054.2004.00332.x
Kleemann, B., Loos, B., Scriba, T. J., Lang, D., Davids, L. M. (2014). St. John’s wort (Hypericum perforatum L.) photomedicine: Hypericin-photodynamic therapy induces metastatic melanoma cell death. PloS One 9, e103762. doi: 10.1371/journal.pone.0103762
Koch, K. G., Chapman, K., Louis, J., Heng-Moss, T., Sarath, G. (2016). Plant tolerance: A unique approach to control hemipteran pests. Front. Plant Sci. 7. doi: 10.3389/fpls.2016.01363
Körner, C. (2016). Plant adaptation to cold climates. F1000Res 5, 2769. doi: 10.12688/f1000research.9107.1
Korn, M., Peterek, S., Mock, H. P., Heyer, A. G., Hincha, D. K. (2008). Heterosis in the freezing tolerance, and sugar and flavonoid contents of crosses between Arabidopsis thaliana accessions of widely varying freezing tolerance. Plant Cell Environ. 31, 813–827. doi: 10.1111/j.1365-3040.2008.01800.x
Kosakivska, I. V. (2008). Biomarkers of plants with different types of ecological strategies. Gen. Appl. Plant Physiol. 34, 113–126.
Kucharíková, A., Kimáková, K., Janfelt, C., Čellárová, E. (2016). Interspecific variation in localization of hypericins and phloroglucinols in the genus Hypericum as revealed by desorption electrospray ionization mass spectrometry imaging. Physiol. Plantarum 157, 2–12. doi: 10.1111/ppl.12422
Kumari, A., Bhinda, M. S., Sharma, S., Chitara, M. K., Debnath, A., Maharana, C., et al. (2021). “ROS regulation mechanism for mitigation of abiotic stress in plants,” in Reactive oxygen species. Ed. Ahmad, R. (London: IntechOpen). doi: 10.5772/intechopen.99845
Kusari, S., Sezgin, S., Nigutová, K., Čellárová, E., Spiteller, M. (2015). Spatial chemo-profiling of hypericin and related phytochemicals in Hypericum species using MALDI-HRMS imaging. Anal. Bioanal. Chem. 407, 4779–4791. doi: 10.1007/s00216-015-8682-6
Latowski, D., Szymańska, R., Strzalka, K. (2014). “Carotenoids involved in antioxidant system of chloroplasts,” in Oxidative damage to plants: Antioxidant networks and signaling. Ed. Ahmad, P. (Amsterdam: Elsevier). doi: 10.1016/B978-0-12-799963-0.00009-5
Lazzara, S., Militello, M., Carrubba, A., Napoli, E., Saia, S. (2017). Arbuscular mycorrhizal fungi altered the hypericin, pseudohypericin, and hyperforin content in flowers of Hypericum perforatum grown under contrasting P availability in a highly organic substrate. Mycorrhiza 27, 345–354. doi: 10.1007/s00572-016-0756-6
Lee, H. Y., Byeon, Y., Back, K. (2014). Melatonin as a signal molecule triggering defense responses against pathogen attack in Arabidopsis and tobacco. J. Pin. Res. 57, 262–268. doi: 10.1111/jpi.12165
Li, Y., Kong, D., Fu, Y., Sussman, M. R., Wu, H. (2020). The effect of developmental and environmental factors on secondary metabolites in medicinal plants. Plant Physiol. Bioch. 148, 80–89. doi: 10.1016/j.plaphy.2020.01.006
Liu, X. N., Zhang, X. Q., Sun, J. S. (2007). Effects of cytokinins and elicitors on the production of hypericins and hyperforin metabololites in Hypericum sampsonii and Hypericum perforatum. Plant Growth Regul. 53, 207–214.
Lu, Y., Chen, Q., Bu, Y., Luo, R., Hao, S., Zhang, J., et al. (2017). Flavonoid accumulation plays an important role in the rust resistance of Malus plant leaves. Front. Plant Sci. 8. doi: 10.3389/fpls.2017.01286
Lukatkin, A. S., Brazaityte, A., Bobinas, C., Duchovskis, P. (2012). Chilling injury in chilling-sensitive plants: A review. Zemdirbyste-Agriculture 99, 111–124.
Makarova, K., Sajkowska-Kozielewicz, J. J., Zawada, K., Olchowik-Grabarek, E., Ciach, M. A., Gogolewski, K., et al. (2021). Harvest time affects antioxidant capacity, total polyphenol and flavonoid content of polish St. John’s wort’s (Hypericum perforatum L.) flowers. Sci. Rep. 11, 3989. doi: 10.1038/s41598-021-83409-4
Manchester, L. C., Coto-Montes, A., Boga, J. A., Andersen, L. P., Zhou, Z., Galano, A., et al. (2015). Melatonin: An ancient molecule that makes oxygen metabolically tolerable. J. Pin. Res. 59, 403–419. doi: 10.1111/jpi.12267
Mañero, F. J. G., Algar, E., Gómez, M. S. M., Sierra, M. D. S., Solano, B. R. (2012). Elicitation of secondary metabolism in Hypericum perforatum by rhizosphere bacteria and derived elicitors in seedlings and shoot cultures. Pharm. Biol. 50, 1–9. doi: 10.3109/13880209.2012.664150
Ma, N., Zhang, J., Reiter, R. J., Ma, X. (2020). Melatonin mediates mucosal immune cells, microbial metabolism, and rhythm crosstalk: A therapeutic target to reduce intestinal inflammation. Med. Res. Rev. 40, 606–632. doi: 10.1002/med.21628
Meirelles, G., Pinhatti, A. V., Sosa-Gomez, D., Rosa, L. M. G., Rech, S. B., von Poser, G. L. (2013). Influence of fungal elicitation with Nomuraea rileyi (Farlow) Samson in the metabolism of acclimatized plants of Hypericum polyanthemum Klotzsech ex Reichardt (Guttiferae). Plant Cell Tiss. Org. 112, 379–385. doi: 10.1007/s11240-012-0234-6
Mhamdi, A., Queval, G., Chaouch, S., Vanderauwera, S., Van Breusegem, F., Noctor, G. (2010). Catalase function in plants: a focus on Arabidopsis mutants as stress-mimic models. J. Exp. Bot. 61, 4197–4220. doi: 10.1093/jxb/erq282
Miller, G., Suzuki, N., Ciftci-Yilmaz, S., Mittler, R. (2010). Reactive oxygen species homeostasis and signalling during drought and salinity stresses. Plant Cell Environ. 33, 453–467. doi: 10.1111/j.1365-3040.2009.02041.x
Miller, G., Suzuki, N., Rizhsky, L., Hegie, A., Koussevitzky, S., Mittler, R. (2007). Double mutants deficient in cytosolic and thylakoid ascorbate peroxidase reveal a complex mode of interaction between reactive oxygen species, plant development, and response to abiotic stresses. Plant Physiol. 144, 1777–1785. doi: 10.1104/pp.107.101436
Mittler, R., Blumwald, E. (2015). The roles of ROS and ABA in systemic acquired acclimation. Plant Cell 27, 64–70. doi: 10.1105/tpc.114.133090
Murch, S. J., Saxena, P. K. (2006). A melatonin-rich germplasm line of St. John’s wort (Hypericum perforatum L.). J. Pin. Res. 41, 284–287. doi: 10.1111/j.1600-079X.2006.00367.x
Nabati, J., Hasanfard, A., Nezami, A., Ahmadi-Lahijani, M. J., Rezazadeh, E. B. (2021). Gas exchange variables as promising criteria for screening freezing-tolerant faba bean (Vicia faba L.) landraces at early growth stages. Legume Science 3, e72. doi: 10.1002/leg3.72
Nabavi, S. M., Šamec, D., Tomczyk, M., Milella, L., Russo, D., Habtemariam, S., et al. (2020). Flavonoid biosynthetic pathways in plants: versatile targets for metabolic engineering. Biotechnol. Adv. 38, 107316. doi: 10.1016/j.biotechadv.2018.11.005
Nadarajah, K. K. (2020). ROS homeostasis in abiotic stress tolerance in plants. Int. J. Mol. Sci. 21, 5208. doi: 10.3390/ijms21155208
Nägele, T., Heyer, A. G. (2013). Approximating subcellular organisation of carbohydrate metabolism during cold acclimation in different natural accessions of Arabidopsis thaliana. New Phytol. 198, 777–787. doi: 10.1111/nph.12201
Namli, S., Işıkalan, C., Akbaş, F., Toker, Z., Tilkat, E. A. (2014). Effects of UV-B radiation on total phenolic, flavonoid and hypericin contents in Hypericum retusum Aucher grown under in vitro conditions. Nat. Prod. Res. 28, 2286–2292. doi: 10.1080/14786419.2014.940588
Nguyen, H. C., Lin, K. H., Ho, S. L., Chiang, C. M., Yang, C. M. (2018). Enhancing the abiotic stress tolerance of plants: From chemical treatment to biotechnological approaches. Physiol. Plantarum 164, 452–466. doi: 10.1111/ppl.12812
Nishimura, T., Zobayed, S. M. A., Kozai, T., Goto, E. (2007). Medicinally important secondary metabolites and growth of Hypericum perforatum L. plants as affected by light quality and intensity. Environ. Control Biol. 45, 113–120. doi: 10.2525/ecb.45.113
Nogueira, T. M. J., Marcelo-Curto, A., Figueiredo, C., Barroso, J. G., Pedro, L. G., Rubiolo, P., et al. (2008). Chemotaxonomy of Hypericum genus from Portugal, geographical distribution and essential oils composition of Hypericum perfoliatum, Hypericum humifusum, Hypericum linarifolium and Hypericum pulchrum. Biochem. Syst. Ecol. 36, 40–50. doi: 10.1016/j.bse.2007.07.004
Nürk, N. M., Madriñán, S., Carine, M. A., Chase, M. W., Blattner, F. R. (2013). Molecular phylogenetics and morphological evolution of St. John’s wort (Hypericum; Hypericaceae). Mol. Phylogenet. Evol. 66, 1–16. doi: 10.1016/j.ympev.2012.08.022
Oberschelp, G., Guarnaschelli, A. B., Teson, N., Harrand, L., Podestá, F. E., Margarit, E. (2020). Cold acclimation and freezing tolerance in three Eucalyptus species: a metabolomic and proteomic approach. Plant Physiol. Bioch. 154, 316–327. doi: 10.1016/j.plaphy.2020.05.026
Odabas, M. S., Camas, N., Çirak, C., Radusiene, J., Janulis, V., Ivanauskas, L. (2010). The quantitative effects of temperature and light intensity on phenolics accumulation in St. John’s wort (Hypericum perforatum). Nat. Prod. Commun. 5, 535–540.
Odabas, M. S., Radusiene, J., Camas, N., Janulis, V., Ivanauskas, L., Çirak, C. (2009). The quantitative effects of temperature and light intensity on hyperforin and hypericins accumulation in Hypericum perforatum L. J. Med. Plants Res. 3, 519–525.
Pagán, I., García-Arenal, F. (2018). Tolerance to plant pathogens: Theory and experimental evidence. Int. J. Mol. Sci. 19, 810. doi: 10.3390/ijms19030810
Panche, A. N., Diwan, A. D., Chandra, S. R. (2016). Flavonoids: An overview. J. Nutr. Sci. 5, e47. doi: 10.1017/jns.2016.41
Pang, Z., Chen, J., Wang, T., Gao, C., Li, Z., Guo, L., et al. (2021). Linking plant secondary metabolites and plant microbiomes: A review. Front. Plant Sci. 12. doi: 10.3389/fpls.2021.621276
Pan, X., Guan, L., Lei, K., Li, J., Zhang, X. (2022). Transcriptional and physiological data revealed cold tolerance in a photo-thermosensitive genic male sterile line Yu17S. BMC Plant Biol. 22, 44. doi: 10.1186/s12870-022-03437-8
Pavlík, M., Vacek, J., Klejdus, B., Kubáň, V. (2007). Hypericin and hyperforin production in St. John’s wort in vitro culture: Influence of saccharose, polyethylene glycol, methyl jasmonate, and Agrobacterium tumefaciens. J. Agr. Food Chem. 55, 6147–6153. doi: 10.1021/jf070245w
Petijová, L., Bruňáková, K., Zámečník, J., Zubrická, D., Mišianiková, A., Kimáková, K., et al. (2014). Relation between frost tolerance and post-cryogenic recovery in Hypericum spp. Cryoletters 35, 171–179.
Petrussa, E., Braidot, E., Zancani, M., Peresson, C., Bertolini, A., Patui, S., et al. (2013). Plant flavonoids–biosynthesis, transport and involvement in stress responses. Int. J. Mol. Sci. 14, 14950–14973. doi: 10.3390/ijms140714950
Porzel, A., Farag, M. A., Mülbradt, J., Wessjohann, L. A. (2014). Metabolite profiling and fingerprinting of Hypericum species: A comparison of MS and NMR metabolomics. Metabolomics 10, 574–588. doi: 10.1007/s11306-013-0609-7
Puijalon, S., Bouma, T. J., Douady, C. J., van Groenendael, J., Anten, N., Martel, E., et al. (2011). Plant resistance to mechanical stress: evidence of an avoidance-tolerance trade-off. New Phytol. 191, 1141–1149. doi: 10.1111/j.1469-8137.2011.03763.x
Qari, S. H., Hassan, M. U., Chattha, M. U., Mahmood, A., Naqve, M., Nawaz, M., et al. (2022). Melatonin induced cold tolerance in plants: Physiological and molecular responses. Front. Plant Sci. 13. doi: 10.3389/fpls.2022.843071
Rahnavard, A., Daneshian, J., Heravan, E., Valadabadi, S., Golein, B. (2012). Investigation of the most important secondary metabolites of St. John’s wort (Hypericum perforatum L.) in Caspian climate. J. Food Agric. Environ. 10, 375–381.
Revuru, B., Bálintová, M., Henzelyová, J., Čellárová, E., Kusari, S. (2020). MALDI-HRMS imaging maps the localization of skyrin, the precursor of hypericin, and pathway intermediates in leaves of Hypericum species. Molecules (Basel). 25, 3964. doi: 10.3390/molecules25173964
Rizzo, P., Altschmied, L., Stark, P., Rutten, T., Gündel, A., Scharfenberg, S., et al. (2019). Discovery of key regulators of dark gland development and hypericin biosynthesis in St. John’s wort (Hypericum perforatum). Plant Biotechnol. J. 17, 2299–2312. doi: 10.1111/pbi.13141
Robson, N. K. B. (1985). “Studies in the genus Hypericum L. (Guttiferae). 3. sections 1. Campylosporus to 6a. Umbraculoides,” in Bulletin of the British museum, vol. 12. (London: British Museum - Natural history), 163–325.
Robson, N. K. B. (1996). “Studies in the genus Hypericum L. (Guttiferae) 6. sections 20. Myriandra to 28. Elodes,” in Bulletin of the natural history museum, vol. 26. (London: The Natural History Museum), 75–217.
Robson, N. K. B. (2001). “Studies in the genus Hypericum L. (Guttiferae). 4(1). sections 7. Roscyna to 9. Hypericum sensu lato (part 1),” in Bulletin of the natural history museum, vol. 31. (London: The Natural History Museum), 37–88.
Robson, N. K. B. (2002). “Studies in the genus Hypericum L. (Guttiferae). 4(2). section 9. Hypericum sensu lato (part 2): subsection 1. Hypericum series 1. Hypericum,” in Bulletin of the natural history museum, vol. 32. (London: The Natural History Museum), 61–123.
Robson, N. K. B. (2006). Studies in the genus Hypericum L. (Clusiaceae). section 9. Hypericum sensu lato (part 3): subsection 1. Hypericum series 2. Senanensia, subsection 2. Erecta and section 9b. Graveolentia. Syst. Biodivers. 4, 19–98. doi: 10.1017/S1477200005001842
Robson, N. K. B. (2010). Studies in the genus Hypericum L. (Hypericaceae) 5(1). sections 10. Olympia to 15/16. Crossophyllum. Phytotaxa 4, 5–126. doi: 10.11646/phytotaxa.4.1.2
Sachdev, S., Ansari, S. A., Ansari, M. I., Fujita, M., Hasanuzzaman, M. (2021). Abiotic stress and reactive oxygen species: Generation, signaling, and defense mechanisms. Antioxidants (Basel). 10, 277. doi: 10.3390/antiox10020277
Saed-Moucheshi, A., Sohrabi, F., Fasihfar, E., Baniasadi, F., Riasat, M., Mozafari, A. A. (2021). Superoxide dismutase (SOD) as a selection criterion for triticale grain yield under drought stress: a comprehensive study on genomics and expression profiling, bioinformatics, heritability, and phenotypic variability. BMC Plant Biol. 21, 148. doi: 10.1186/s12870-021-02919-5
Saffariha, M., Jahani, A., Jahani, R., Latif, S. (2021). Prediction of hypericin content in Hypericum perforatum L. in different ecological habitat using artificial neural networks. Plant Methods 17, 10. doi: 10.1186/s13007-021-00710-z
Sahoo, R., Kumar, S., Ahuja, P. S. (2001). Induction of a new isozyme superoxide dismutase at low temperature in Potentilla astrisanguinea Lodd. variety argyrophylla (Wall.ex. Lehm) Griers. J. Plant Physiol. 158, 1093–1097. doi: 10.1078/0176-1617-00224
Schenke, D., Utami, H. P., Zhou, Z., Gallegos, M. T., Cai, D. (2019). Suppression of UV-B stress induced flavonoids by biotic stress: Is there reciprocal crosstalk? Plant Physiol. Bioch. 134, 53–63. doi: 10.1016/j.plaphy.2018.06.026
Schulz, E., Tohge, T., Winkler, J. B., Albert, A., Schoffner, A. R., Fernie, A. R., et al. (2021). Natural variation among Arabidopsis accessions in the regulation of flavonoid metabolism and stress gene expression by combined UV radiation and cold. Plant Cell Physiol. 62, 502–514. doi: 10.1093/pcp/pcab013
Schulz, E., Tohge, T., Zuther, E., Fernie, A. R., Hincha, D. K. (2015). Natural variation in flavonol and anthocyanin metabolism during cold acclimation in Arabidopsis thaliana accessions. Plant Cell Environ. 38, 1658–1672. doi: 10.1111/pce.12518
Schulz, E., Tohge, T., Zuther, E., Fernie, A. R., Hincha, D. K. (2016). Flavonoids are determinants of freezing tolerance and cold acclimation in Arabidopsis thaliana. Sci. Rep. 6, 34027. doi: 10.1038/srep34027
Sen Raychaudhuri, S., Deng, X. W. (2000). The role of superoxide dismutase in combating oxidative stress in higher plants. Bot. Rev. 66, 89–98. doi: 10.1007/BF02857783
Sewelam, N., Kazan, K., Schenk, P. M. (2016). Global plant stress signaling: Reactive oxygen species at the cross-road. Front. Plant Sci. 7, 187. doi: 10.3389/fpls.2016.00187
Sewelam, N., Oshima, Y., Mitsuda, N., Ohme-Takagi, M. (2014). A step towards understanding plant responses to multiple environmental stresses: a genome-wide study. Plant Cell Environ. 37, 2024–2035. doi: 10.1111/pce.12274
Shah, A., Smith, D. L. (2020). Flavonoids in agriculture: Chemistry and roles in, biotic and abiotic stress responses, and microbial associations. Agronomy 10, 1209. doi: 10.3390/agronomy10081209
Sharafi, E., Nekoei, S. M., Fotokian, M. H., Davoodi, D., Mirzaei, H. H., Hasanloo, T. (2013). Improvement of hypericin and hyperforin production using zinc and iron nano-oxides as elicitors in cell suspension culture of St john's wort (Hypericum perforatum L.). JMPB 2, 177–184.
Sharma, P., Jha, A. B., Dubey, R. S., Pessarakli, M. (2012). Reactive oxygen species, oxidative damage, and antioxidative defense mechanism in plants under stressful conditions. J. Bot. 2012, 217037. doi: 10.1155/2012/217037
Singh, R. K., Hou, W., Marslin, G., Dias, A., C., P., Franklin, G. (2014). Lignin and flavonoid content increases in Hypericum perforatum cell wall after Agrobacterium tumefaciens co-cultivation. Planta Med. 80, P1M22. doi: 10.1055/s-0034-1394589
Sirvent, T. M., Gibson, D. (2002). Induction of hypericins and hyperforin in Hypericum perforatum L. in response to biotic and chemical elicitors. Physiol. Mol. Plant P. 60, 311–320. doi: 10.1006/pmpp.2002.0410
Sirvent, T. M., Krasnoff, S. B., Gibson, D. M. (2003). Induction of hypericins and hyperforins in Hypericum perforatum in response to damage by herbivores. J. Chem. Ecol. 29, 2667–2681. doi: 10.1023/b:joec.0000008011.77213.64
Skyba, M., Petijová, L., Košuth, J., Koleva, D. P., Ganeva, T. G., Kapchina-Toteva, V. M., et al. (2012). Oxidative stress and antioxidant response in Hypericum perforatum L. plants subjected to low temperature treatment. J. Plant Physiol. 169, 955–964. doi: 10.1016/j.jplph.2012.02.017
Skyba, M., Urbanová, M., Kapchina-Toteva, V., Košuth, J., Harding, K., Čellárová, E. (2010). Physiological, biochemical and molecular characteristics of cryopreserved Hypericum perforatum L. shoot tips. Cryo Letters 31, 249–260.
Soliman, W. S., Sugiyama, S., Abbas, A. M. (2018). Contribution of avoidance and tolerance strategies towards salinity stress resistance in eight C3 turfgrass species. Hortic. Environ. Biote. 59, 29–36. doi: 10.1007/s13580-018-0004-4
Soltabayeva, A., Ongaltay, A., Omondi, J. O., Srivastava, S. (2021). Morphological, physiological and molecular markers for salt-stressed plants. Plants (Basel). 10, 243. doi: 10.3390/plants10020243
Sooriamuthu, S., Varghese, R. J., Bayyapureddy, A., John, S. S. T., Narayanan, R. (2013). Light-induced production of antidepressant compounds in etiolated shoot cultures of Hypericum hookerianum Wight & Arn. (Hypericaceae). Plant Cell Tiss. Org. 115, 169–178. doi: 10.1007/s11240-013-0350-y
Southwell, I. A., Bourke, C. A. (2001). Seasonal variation in hypericin content of Hypericum perforatum L. (St. John’s Wort). Phytochemistry 56, 437–441. doi: 10.1016/s0031-9422(00)00411-8
Soydam Aydin, S., Büyük, I., Aras, S. (2013). Relationships among lipid peroxidation, SOD enzyme activity, and SOD gene expression profile in Lycopersicum esculentum L. exposed to cold stress. Genet. Mol. Res. 12, 3220–3229. doi: 10.4238/2013.August.29.6
Squeo, F. A., Rada, F., Azocar, A., Goldstein, G. (1991). Freezing tolerance and avoidance in high tropical Andean plants: Is it equally represented in species with different plant height? Oecologia 86, 378–382. doi: 10.1007/BF00317604
Stazi, M., Negro, S., Megighian, A., D'Este, G., Solimena, M., Jockers, R., et al. (2021). Melatonin promotes regeneration of injured motor axons via MT1 receptors. J. Pineal Res. 70, e12695. doi: 10.1111/jpi.12695
Stoyanova-Koleva, D., Stefanova, M., Ganeva, T. S., Čellárová, E. (2015). Structural modifications in the mesophyll associated with cryopreservation of seven Hypericum species. Biol. Plantarum. 59, 514–520. doi: 10.1007/s10535-015-0528-8
Sung, D. Y., Kaplan, F., Lee, K. J., Guy, C. L. (2003). Acquired tolerance to temperature extremes. Trends Plant Sci. 8, 179–187. doi: 10.1016/S1360-1385(03)00047-5
Swiderski, A., Muras, P., Koloczek, H. (2004). Flavonoid composition in frost-resistant Rhododendron cultivars grown in Poland. Sci. Hortic-Amsterdam. 100, 139–151. doi: 10.1016/j.scienta.2003.08.013
Sytar, O., Kotta, K., Valasiadis, D., Kosyan, A., Brestic, M., Koidou, V., et al. (2021). The effects of photosensitizing dyes fagopyrin and hypericin on planktonic growth and multicellular life in budding yeast. Molecules (Basel). 26, 4708. doi: 10.3390/molecules26164708
Szymański, J., Bocobza, S., Panda, S., Sonawane, P., Cárdenas, P. D., Lashbrooke, J., et al. (2020). Analysis of wild tomato introgression lines elucidates the genetic basis of transcriptome and metabolome variation underlying fruit traits and pathogen response. Nat. Genet. 52, 1111–1121. doi: 10.1038/s41588-020-0690-6
Tattini, M., Galardi, C., Pinelli, P., Massai, R., Remorini, D., Agati, G. (2004). Differential accumulation of flavonoids and hydroxycinnamates in leaves of Ligustrum vulgare under excess light and drought stress. New Phytol. 163, 547–561. doi: 10.1111/j.1469-8137.2004.01126.x
Theodossiou, T. A., Olsen, C. E., Jonsson, M., Kubin, A., Hothersall, J. S., Berg, K. (2017). The diverse roles of glutathione-associated cell resistance against hypericin photodynamic therapy. Redox Biol. 12, 191–197. doi: 10.1016/j.redox.2017.02.018
Thomashow, M. F. (1999). Plant cold acclimation: Freezing tolerance genes and regulatory mechanisms. Annu. Rev. Plant Biol. 50, 571–599. doi: 10.1146/annurev.arplant.50.1.571
Tusevski, O., Stanoeva, J. P., Stefova, M., Gadzovska-Simic, S. (2015). Agrobacterium enhances xanthone production in Hypericum perforatum cell suspensions. Plant Growth Regul. 76, 199–210. doi: 10.1007/s10725-014-9989-6
Tusevski, O., Vinterhalter, B., Krstić Milošević, D., Soković, M., Ćirić, A., Vinterhalter, D., et al. (2017). Production of phenolic compounds, antioxidant and antimicrobial activities in hairy root and shoot cultures of Hypericum perforatum L. Plant Cell Tiss. Org. 128, 589–605. doi: 10.1007/s11240-016-1136-9
Uarrota, V. G., Stefen, D. L. V., Leolato, L. S., Gindri, D. M., Nerling, D. (2018). “Revisiting carotenoids and their role in plant stress responses: from biosynthesis to plant signaling mechanisms during stress,” in Antioxidants and antioxidant enzymes in higher plants. Eds. Gupta, D., Palma, J., Corpas, F. (Cham: Springer), 207–232. doi: 10.1007/978-3-319-75088-0_10
Valizadeh, R., Toorchi, M., Mogadam, M., Mohammadi, H., Pessarakli, M. (2017). Effects of freeze and cold stress on certain physiological and biochemical traits in sensitive and tolerant barley (Hordeum vulgare) genotypes. J. Plant Nutr. 41, 102–111. doi: 10.1080/01904167.2017.1381730
van Buer, J., Cvetkovic, J., Baier, M. (2016). Cold regulation of plastid ascorbate peroxidases serves as a priming hub controlling ROS signaling in Arabidopsis thaliana. BMC Plant Biol. 16, 163. doi: 10.1186/s12870-016-0856-7
Vanderauwera, S., Suzuki, N., Miller, G., van de Cotte, B., Morsa, S., Ravanat, J. L., et al. (2011). Extranuclear protection of chromosomal DNA from oxidative stress. PNAS 108, 1711–1716. doi: 10.1073/pnas.1018359108
Verbruggen, N., Hermans, C. (2008). Proline accumulation in plants: a review. Amino Acids 35, 753–759. doi: 10.1007/s00726-008-0061-6
Wang, Y., Jiang, Y., Zou, M., Liu, J., Zhao, H., Wang, Y. (2022). Antidepressant actions of melatonin and melatonin receptor agonist: Focus on pathophysiology and treatment. Behav. Brain Res. 420, 113724. doi: 10.1016/j.bbr.2021.113724
Wang, J., Qian, J., Yao, L., Lu, Y. (2015). Enhanced production of flavonoids by methyl jasmonate elicitation in cell suspension culture of Hypericum perforatum. Bioresour. Bioprocess 2, 5. doi: 10.1186/s40643-014-0033-5
Wasternack, C., Strnad, M. (2019). Jasmonates are signals in the biosynthesis of secondary metabolites – pathways, transcription factors and applied aspects – a brief review. New Biotechnol. 48, 1–11. doi: 10.1016/j.nbt.2017.09.007
Wei, Y., Chen, H., Wang, L., Zhao, Q., Wang, D., Zhang, T. (2022). Cold acclimation alleviates cold stress-induced PSII inhibition and oxidative damage in tobacco leaves. Plant Signal. Behav. 17, 2013638. doi: 10.1080/15592324.2021.2013638
Wise, R. R., Naylor, A. W. (1987). Chilling-enhanced photooxidation: The peroxidative destruction of lipids during chilling injury to photosynthesis and ultrastructure. Plant Physiol. 83, 272–277. doi: 10.1104/pp.83.2.272
Xu, Y., Fu, X. (2022). Reprogramming of plant central metabolism in response to abiotic stresses: A metabolomics view. Int. J. Mol. Sci. 23, 5716. doi: 10.3390/ijms23105716
Xu, L., Zhang, M., Zhang, X., Han, L. (2015). Cold acclimation treatment–induced changes in abscisic acid, cytokinin, and antioxidant metabolism in zoysiagrass (Zoysia japonica). Hort Sci. 50, 1075–1080. doi: 10.21273/HORTSCI.50.7.1075
Yadav, A. K., Carroll, A. J., Estavillo, G. M., Rebetzke, G. J., Pogson, B. J. (2019). Wheat drought tolerance in the field is predicted by amino acid responses to glasshouse-imposed drought. J. Exp. Bot. 70, 4931–4948. doi: 10.1093/jxb/erz224
Yamaner, O., Erdag, B. (2013). Effects of sucrose and polyethylene glycol on hypericins content in Hypericum adenotrichum. Eurasia J. Biosci. 7, 101–110. doi: 10.5053/ejobios.2013.7.0.12
Yang, X., Cushman, J. C., Borland, A. M., Liu, Q. (2020). Editorial: Systems biology and synthetic biology in relation to drought tolerance or avoidance in plants. Front. Plant Sci. 11. doi: 10.3389/fpls.2020.00394
Yang, L., Wen, K. S., Ruan, X., Zhao, Y. X., Wei, F., Wang, Q. (2018). Response of plant secondary metabolites to environmental factors. Molecules (Basel). 23, 762. doi: 10.3390/molecules23040762
Yang, Z., Wu, Y., Li, Y., Ling, H. Q., Chu, C. (2009). OsMT1a, a type 1 metallothionein, plays the pivotal role in zinc homeostasis and drought tolerance in rice. Plant Mol. Biol. 70, 219–229. doi: 10.1007/s11103-009-9466-1
Yao, Y., Kang, T., Jin, L., Liu, Z., Zhang, Z., Xing, H., et al. (2019). Temperature-dependent growth and hypericin biosynthesis in Hypericum perforatum. Plant Physiol. Bioch. 139, 613–619. doi: 10.1016/j.plaphy.2019.04.012
Yin, L., Wang, S., Eltayeb, A. E., Uddin, M. I., Yamamoto, Y., Tsuji, W., et al. (2010). Overexpression of dehydroascorbate reductase, but not monodehydroascorbate reductase, confers tolerance to aluminum stress in transgenic tobacco. Planta 231, 609–621. doi: 10.1007/s00425-009-1075-3
You, J., Chan, Z. (2015). ROS regulation during abiotic stress responses in crop plants. Front. Plant Sci. 6. doi: 10.3389/fpls.2015.01092
Zambounis, A., Sytar, O., Valasiadis, D., Hilioti, Z. (2020). Effect of photosensitisers on growth and morphology of Phytophthora citrophthora coupled with leaf bioassays in pear seedlings. Plant Protect. Sci. 56, 74–82. doi: 10.17221/102/2019-pps
Zandalinas, S. I., Fichman, Y., Devireddy, A. R., Sengupta, S., Azad, R. K., Mittler, R. (2020). Systemic signaling during abiotic stress combination in plants. PNAS 117, 13810–13820. doi: 10.1073/pnas.2005077117
Zdunić, G., Gođevac, D., Savikin, K., Petrovic, S. (2017). Comparative analysis of phenolic compounds in seven Hypericum species and their antioxidant properties. Nat. Prod. Commun. 12, 1805–1811. doi: 10.1177/1934578X1701201140
Zhang, Q., Zhai, J., Shao, L., Lin, W., Peng, C. (2019). Accumulation of anthocyanins: an adaptation strategy of Mikania micrantha to low temperature in winter. Front. Plant Sci. 10. doi: 10.3389/fpls.2019.01049
Zhen, Y., Ungerer, M. C. (2008). Clinal variation in freezing tolerance among natural accessions of Arabidopsis thaliana. New Phytol. 177, 419–427. doi: 10.1111/j.1469-8137.2007.02262.x
Zhou, W., Yang, S., Zhang, Q., Xiao, R., Li, B., Wang, D., et al. (2021). Functional characterization of serotonin n-acetyltransferase genes (SNAT1/2) in melatonin biosynthesis of Hypericum perforatum. Front. Plant Sci. 12. doi: 10.3389/fpls.2021.781717
Zisapel, N. (2018). New perspectives on the role of melatonin in human sleep, circadian rhythms and their regulation. Brit. J. Pharmacol. 175, 3190–3199. doi: 10.1111/bph.14116
Zobayed, S. M. A., Afreen, F., Goto, E., Kozai, T. (2006). Plant-environment interactions: Accumulation of hypericin in dark glands of Hypericum perforatum. Ann. Bot. 98, 793–804. doi: 10.1093/aob/mcl169
Zobayed, S. M. A., Afreen, F., Kozai, T. (2005). Temperature stress can alter the photosynthetic efficiency and secondary metabolite concentrations in St. John’s wort. Plant Physiol. Bioch. 43, 977–984. doi: 10.1016/j.plaphy.2005.07.013
Zobayed, S. M. A., Murch, S. J., Rupasinge, H. P. V., Saxena, P. K. (2003). Elevated carbon supply altered hypericin and hyperforin contents of St. John’s wort (Hypericum perforatum) grown in bioreactors. Plant Cell Tiss. Org. 75, 143–149. doi: 10.1023/A:1025053427371
Keywords: Phenolic compounds, proline, carotenoids, CAT, SOD, APX, cold stress
Citation: Bruňáková K, Bálintová M, Petijová L and Čellárová E (2022) Does phenotyping of Hypericum secondary metabolism reveal a tolerance to biotic/abiotic stressors? Front. Plant Sci. 13:1042375. doi: 10.3389/fpls.2022.1042375
Received: 12 September 2022; Accepted: 24 October 2022;
Published: 30 November 2022.
Edited by:
Carla Pinheiro, New University of Lisbon, PortugalReviewed by:
Fatih Seyis, Recep Tayyip Erdoğan University, TurkeyOksana Sytar, Taras Shevchenko National University of Kyiv, Ukraine
Copyright © 2022 Bruňáková, Bálintová, Petijová and Čellárová. This is an open-access article distributed under the terms of the Creative Commons Attribution License (CC BY). The use, distribution or reproduction in other forums is permitted, provided the original author(s) and the copyright owner(s) are credited and that the original publication in this journal is cited, in accordance with accepted academic practice. No use, distribution or reproduction is permitted which does not comply with these terms.
*Correspondence: Katarína Bruňáková, katarina.brunakova@upjs.sk
†These authors have contributed equally to this work and share first authorship