Defensive patterns of chestnut genotypes (Castanea spp.) against the gall wasp, Dryocosmus kuriphilus
- 1Unit for Sustainable Forest and Environmental Management, University of Santiago de Compostela, Lugo, Spain
- 2Department of Biological Sciences, Dartmouth College, Hanover, NH, United States
- 3Department of Organisms and Systems Biology, Polytechnic School of Mieres, University of Oviedo, Mieres, Spain
- 4Department of Engineering and Agricultural Sciences, School of Agricultural and Forestry Engineering, University of León, León, Spain
Plants draw from various genetically controlled defenses to protect against herbivores and pathogens. The efficacy of alternative defenses can vary with the biology and phylogeny of the enemy. Dryocosmus kuriphilus, an invasive gall maker native to China, has become one of the main pests of chestnut trees around the world. We studied genetic variation in resistance and susceptibility to D. kuriphilus within a replicated blocked planting of 12 chestnut genotypes established in Galicia, Spain in 2004. We found very high genetic variation in susceptibility to D. kuriphilus. We evaluated if the variation was due to host selection by the wasp, differential efficacy of inducible defenses in the trees, or variability in susceptibility of the plant to manipulation by the gallmaker. We assessed host selection by counting number of eggs laid by females in tree buds and comparing preferences with phytochemistry. We also measured inducible changes in phytochemistry within and around galls, gall physical characteristics, parasitism, and insect fitness. The effective defense mechanisms in resistant genotypes involved (1) hypersensitive reactions to eggs or neonates within buds and (2) early precise abscission of nascent galls from expanding leaves. Surprisingly, the genetic resistance to D. kuriphilus of the chestnut genotypes we tested was not related to phenols, terpenes, and primary nutrition.
Introduction
Plants protect themselves against insects and pathogens with a combination of chemical and physical barriers, which are either constitutive or induced by the insect or pathogen attack (Karban and Baldwin, 1997; Wagner et al., 2002; Franceschi et al., 2005; Barto et al., 2008).
Physical defenses are made of structural elements that provide toughness or thickness to the tissues and may involve waxes, trichomes, spines, thorns, and lignification. Chemical defenses can include secondary metabolites, such as terpenes, tannins, and phenolic compounds (Azarkan et al., 2004; Van Zandt and Agrawal, 2004), and defensive proteins (Swapan and Muthukrishnan, 1999; Ekramoddoullah et al., 2000; Lombardero et al., 2016). Secondary metabolites can affect herbivorous insects and pathogens directly, through antifeedant and toxic effects, or indirectly, through the attraction of natural enemies (Pearce, 1996; Wagner et al., 2002; War et al., 2012).
Some induced plant defenses serve to limit the extent of damage by an attacking enemy (Franceschi et al., 2005). One class is known as hypersensitive response (HR) (Fernandes, 1998), which have been recognized as a plant defense mechanism since the early 20th century (Ward, 1902). HR is characterized by a genetically controlled progression of cell death in the host tissue at the point of infection (Dangl and Jones, 2001; Bleiker and Uzunovic, 2004). The process typically involves production of reactive oxygen species that kill the host cells and (often) the invading organisms. HR have been most frequently described for fungi, bacteria, and viruses (Franceschi et al., 2005), but have also been reported for some insects with particularly intimate relationships with host tissue, such as leaf miners and gall makers (Fernandes, 1990; Walling, 2000; Ollerstam et al., 2002; Kaloshian, 2004).
Another localized inducible defense is the production of histological defensive structures such as cork layers beyond the infection point, abscission layers between diseased and lignified healthy areas, and tyloses in xylem vessels (Agrios, 2005; Park and Kim, 2019). Abscission is a cell separation process by which plants can drop some of their organs. It is the consequence of a breakdown in adhesion between highly differentiated cells at the site of organ shedding (the abscission zone, Roberts et al., 2002). The abscission zone marks the line beyond which all of the more distal cells and tissue will be separated from the plant (Patterson, 2001). Abscission of infested leaves, fruits, or seeds is common (Boucher and Sork, 1979; Strauss and Zangerl, 2002) and is considered especially effective against insects with low mobility (Faeth et al., 1981; Stiling et al., 1991; Connor and Taverner, 1997). For instance, in Rhus glabra leaf abscission is ten times higher on leaves infected by the gall maker Melaphis rhois (Homoptera: Aphididae) than on ungalled leaves (Fernandes, 1999) and premature acorn abscission had negative consequences for Curculio elephas Gyll. (Coleoptera: Curculionidae) by reducing larval size (Bonal and Muñoz, 2008).
Generally, these defensive reactions are regulated by three phytohormones; jasmonic acid (JA) and ethylene (ET) tend to elicit defenses against herbivores and necrotrophic pathogens (Kessler and Baldwin, 2002; Thaler et al., 2012), whereas salicylic acid (SA) is mainly involved in defense against phloem-sap-sucking insects and biotrophic pathogens (Spoel et al., 2007; Zhang et al., 2009). SA plays an important role regulating programmed cell dead (Vlot et al., 2009).
Galling insects are defined by their capacity to modify plant physiology such that there is regulated development of complex gall structures in the plant tissue (Harper et al., 2004). Gall tissues then provide food and physical protection for the larvae developing within them (Cornell, 1983; Price et al., 1987; Stone and Schönrogge, 2003; Bailey et al., 2009).
The ovipositing females of galling insects have been reported to select host plants based on plant morphology, phenology, vigor (Anderson et al., 1989; Eliason and Potter, 2000; Pires and Price, 2000; Yukawa, 2000), and chemistry (Abrahamson et al., 2003; Naidoo et al., 2018). Host chemistry is also important for larval performance and can trigger host shifts (Rehill and Schultz, 2012; Kot et al., 2018).
Inducible defenses in plants are thought to be especially important for galling insects because of their intimate relationship with plant tissue (Cornell, 1983; Hartley, 1998; Abrahamson et al., 2003). HR has been described as a common resistance strategy against gall-inducers (Fernandes, 1990; Abrahamson et al., 1991; Fernandes et al., 2000; Fernandes and Negreiros, 2001). The sessile habit of the galls may also provide opportunity for the host plant to respond with a premature abscission of galled leaves although this mechanism is less studied (Williams and Whitham, 1986; Fernandes et al., 2008). Some insect species appear to adaptively induce gall abscission when their feeding is completed, but an early gall abscission can cause larval mortality (Fernandes et al., 2008).
Galling insects benefit from controlling both the physical and chemical aspects of the plant tissue within which they reside, as they can manipulate plant defenses to their own advantage (Schultz, 1988; Hartley, 1998; Oliveira et al., 2016; Kot et al., 2018). However, inducible changes in plant tissue within developing galls can work for or against the galling insect; patterns could vary depending upon the class of secondary metabolites. Inducible increases in terpenes in galled plant tissue might have direct toxic effects on the attacking insect or attract parasitoids (both bad for the galling insect), but could also serve as oviposition cues or to defend the attacking insect from its own enemies (good for the galling insect) (Rostas et al., 2013; Naidoo et al., 2018). The role of phenolic compounds is also ambiguous. Some studies show that phenolic compounds are unrelated to host plant resistance (Abrahamson et al., 1991), while others indicate the opposite (Westphal et al., 1981). Galls may contain lower (Nyman and Julkunen-Tiitto, 2000; Allison and Schultz, 2005) or higher (Hartley, 1998) phenolic concentration compared with ungalled tissue, and the adaptive value of these chemical alterations is uncertain (Inbar et al., 2010). Tannins can be dramatically elevated in galled plant tissue. Galls from cynipid wasps in oak trees contain such high concentrations that they have been used as commercial sources of pure tannin (Cornell, 1983). Tannins sometimes reduce the growth and survival of insect herbivores (Bernays et al., 1980; Karowe, 1989; Ayres et al., 1997; Nomura and Itioka, 2002). Conversely, high tannin concentrations in galls might protect the larvae against damaging agents (Cornell, 1983; Taper et al., 1986; Schultz, 1992).
Dryocosmus kuriphilus Yasumatsu (Hymenoptera, Cynipidae) is an invasive gall maker, native to China that has become one of the main pest of chestnut trees around the world (EPPO, 2005). Dryocosmus kuriphilus was detected in Europe in 2002 (Brussino et al., 2002) and spread quickly through the chestnut stands compromising chestnut production (Quacchia et al., 2008). Parthenogenic females lay eggs in the tree buds during the summer. The number of eggs per female can exceed 120 eggs (Lombardero et al., 2021) and are laid by the females in small groups. Eggs and the first instar larvae overwinter within the buds. In the following spring, gall formation interferes with the normal development of vegetative and reproductive structures, reduces leaf area, and reduces the formation of female flowers, which collectively lead to a reduction in wood and nut production (Kato and Hijii, 1997; Battisti et al., 2014; Marcolin et al., 2021). D. kuriphilus recruits natural enemies from native Cynipidae in all the areas where it has been introduced, although the potential for native parasitoids to regulate D. kuriphilus populations has been questioned (Quacchia et al., 2013; Panzavolta et al., 2018; Gil-Tapetado et al., 2021).
Castanea species (Fagaceae) are important forest trees in Europe, Asia, and North America. Global chestnut production has increased continuously over the last 40 years (Freitas et al., 2021). European chestnut, Castanea sativa Miller, grows mainly in southern and western Europe and there is a long tradition of cultivation for fruit and wood (Conedera et al., 2004). Chestnuts cover an area of more than 100,000 ha in northwestern Spain, of which about 45,000 ha are in Galicia (MARM, 2011), where this study was conducted. Spain is considered the second (Fernandes et al., 2022) or third (Freitas et al., 2021) largest producer of chestnuts in the world. The European chestnut has no native fauna of gall makers, but other members of Fagaceae (especially Quercus spp.) support a rich fauna of cynipid gall wasps in Europe (Stone et al., 2002).
Castanea sativa in NW Spain has been seriously affected by Phytophthora spp. for the last two centuries (Alcaide et al., 2020; Fernandes et al., 2022). Since some Asian species of Castanea were considered resistant to the disease, a program of hybridization between European chestnut and two Asian chestnut species (C. crenata Siebold and Zucc. and C. mollissima Blume) was started, with the aim of producing hybrids resistant to the disease (Fernández-López, 2011). This hybrid material is being used in clonal forestry for wood and nut production and as rootstocks for grafting with traditional varieties. These hybrid clones show high variation in susceptibility to D. kuriphilus (Míguez-Soto et al., 2018; Castedo-Dorado et al., 2021). Little is known about the defensive patterns of chestnuts against D. kuriphilus, but HR was previously reported for one chestnut hybrid (Dini et al., 2012) and in a chestnut cultivar ecotype (Nugnes et al., 2018).
Dryocosmus kuriphilus was detected in Galicia in 2014 (Nieves-Aldrey et al., 2019) and since them spread quickly through the territory (Gil-Tapetado et al., 2021). In 2015, the wasp started to colonize a field trial established with hybrids of the European and Asian chestnut as well as the parental species. The different genotypes present in the trial showed great variability in terms of their susceptibility to D. kuriphilus (Castedo-Dorado et al., 2021).
We sought to evaluate three alternative hypotheses for this variability: (H1) differences in susceptibility are due to the host selection by the gall wasp; (H2) different genotypes show different efficacy of inducible defenses against the wasp; and (H3) the susceptible genotypes are those that insects manipulate most successfully.
Materials and methods
Field trial and experimental design
The study was carried out in a trial located in San Xoan de Lagostelle (Galicia, NW Spain), at an elevation of 505 m a.s.l. (UTM Coordinates: 587161 m E, 4786989 m N; ETRS89 H29T). The trial was established in spring 2004 to test certain traits of interest in the Galician breeding program for hybrid chestnut clones (Castedo-Dorado et al., 2021). Dryocosmus kuriphilus colonized the planting in 2015, which coincides with the beginning of our study.
The trial was a randomized complete block design of 30 hybrid clones of Castanea sativa × C. crenata, one hybrid of C. sativa × C. mollissima, and the three pure species (C. sativa, C. crenata, and C. mollissima). Native Castanea sativa is known to express different degrees of susceptibility to D. kuriphilus (Sartor et al., 2009). The Japanese species C. crenata is highly susceptible to the wasp (Murakami, 2010), but some resistant varieties were also described (Shimura, 1972). Castanea mollissima, the Chinese chestnut, shares range with the invasive wasp and is regarded as susceptible to this species (EPPO, 2005). Each block included seven individuals of each clones, and each block was replicated six times. The trial was established at the bottom of a shallow valley, with four blocks in shady exposure and two in sunshine. We selected the four shady replicates for this study, to minimize variation due to topography and geographic orientation (Figure 1). The different genotypes showed high variability in susceptibility to D. kuriphilus, with seven clones showing total resistance after 4 years of attack (i.e., no galls in the crown in mid-summer) while others showed medium or high attack levels (see Castedo-Dorado et al., 2021). However, when visiting the trial in spring to collect leaves for other studies, we observed that some of these resistant clones showed small galls in leaves that, somehow, disappeared later (Figure 2). This motivated our studies of leaf chemistry (terpenes, total phenols, and condensed tannins) as it related to susceptibility to D. kuriphilus. At the same time, we wanted to test if leaf chemistry played some role in tree selection by the insect to lay its eggs.
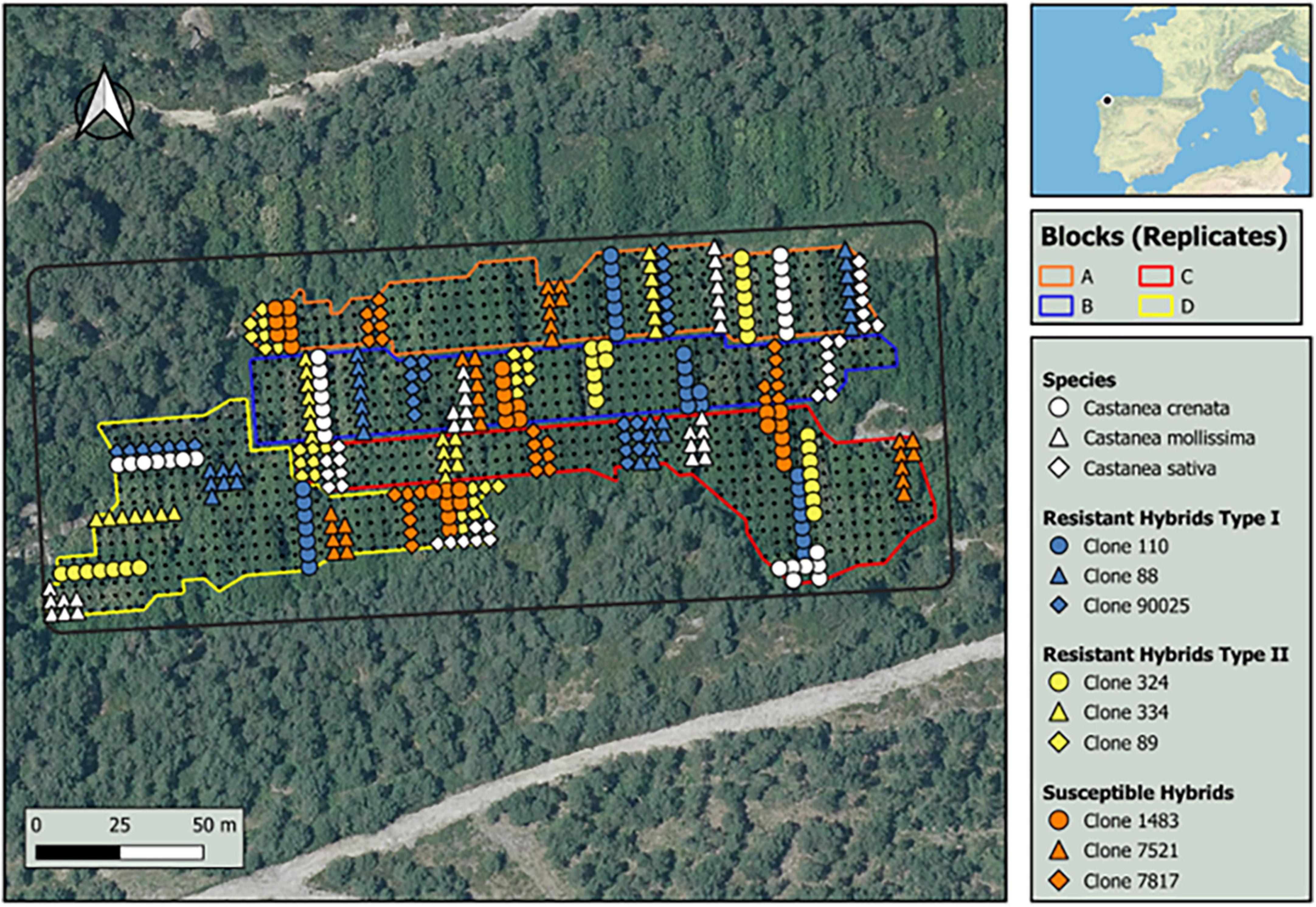
Figure 1. Location of the field trial in Galicia (NW of Spain), and of the selected genotypes within the four replicates.
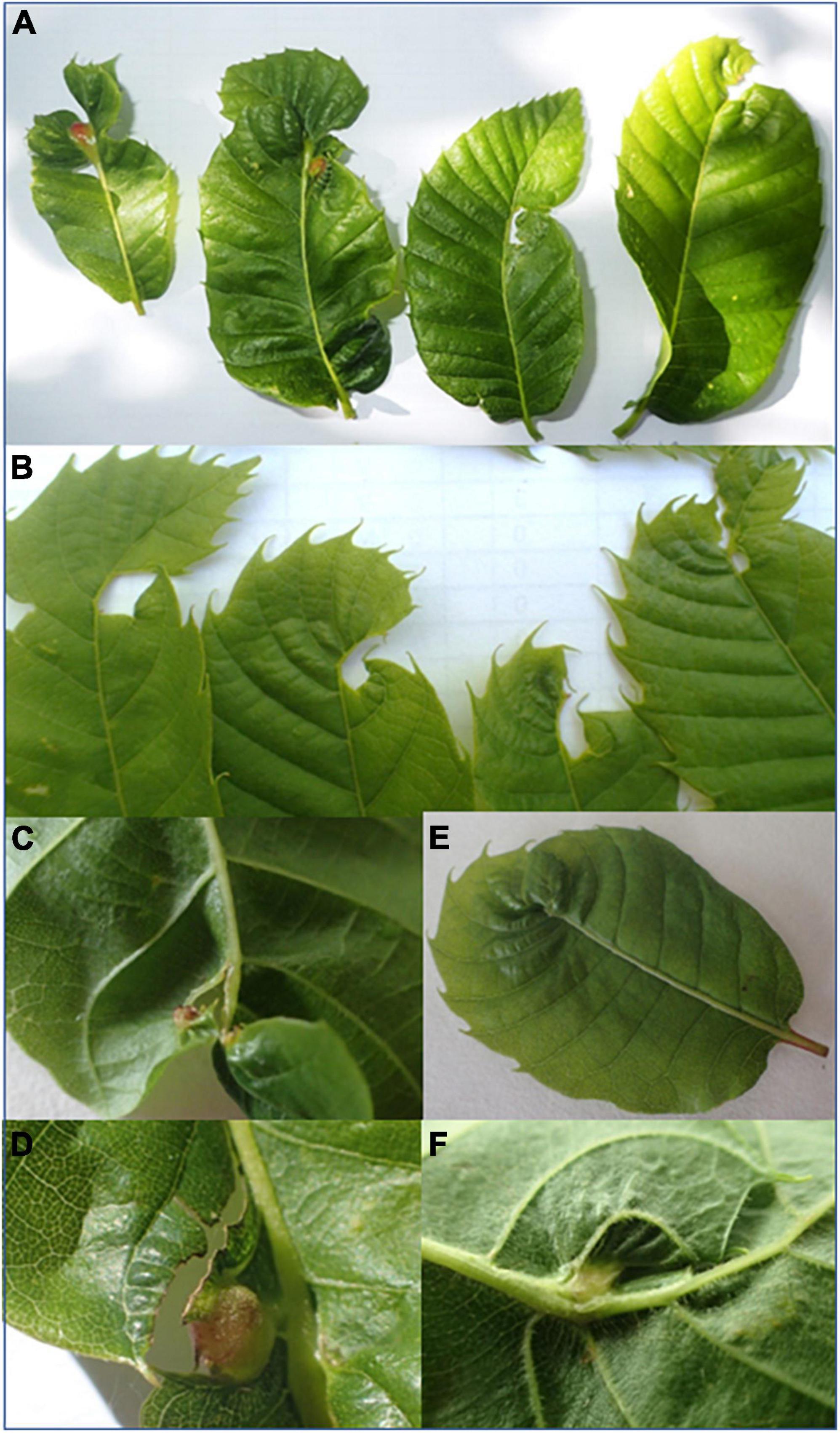
Figure 2. Spring leaves of Resistant Type I: (A) Small galls in the leaves (left) and empty areas after gall drop (right). (B) Close up of abscised gall zones. (C,D) Gall detaching from the leave (gall abscission). (E,F) Deformed leave with small galls without larvae inside.
For the current study, we selected the three chestnut species (C. sativa, C. crenata, and C. mollissima) and nine hybrid chestnut clones from the four replications (Figure 1). The nine hybrid chestnuts were classified in relation to its susceptibility to D. kuriphilus. Three clones were considered “susceptible” (clone ‘1483,’ clone ‘7521,’ and clone ‘7817’), i.e., showed high attack levels of D. kuriphilus based in the results of attack levels obtained in Castedo-Dorado et al. (2021) and six were considered resistant. The resistant clones were further divided into two types. Type I resistance was defined as those resistant clones that harbored some leaf galls in the beginning of the season but disappeared later (clone ‘88’, clone ‘110’, and clone ‘90025’). Type II resistance was defined as those clones with no visible galls (clone ‘89’, clone ‘324’, and clone ‘334’). All hybrids were obtained by crossing C. crenata and C. sativa, except the susceptible clone ‘7521’ that was obtained from C. mollissima × C. sativa.
Field and laboratory sampling
In all study trees, we measured diameter at breast height, tree height, and total number of galls in the crown. Tree size was measured in March 2018 with a caliper and digital hypsometer. Galls were counted in July of 2016–2018 by the same observers (ML and FC-D), using binoculars as needed. For this study, we only considered attacks in 2018, by which time D. kuriphilus was fully distributed across the area of the trial (Castedo-Dorado et al., 2021).
On 1 July 2019, we collected 312 galls (23–51 per clone). In the laboratory, we measured the diameter of each gall in three perpendicular planes to estimate gall volume assuming the shape of an ellipse. Then the galls were dissected to count number of cells (each cell representing one initial egg), number of D. kuriphilus larvae and the presence of ectoparasites. The native ectoparasitoids were in the larval stage, so we did not identify them to species, and the percentage of parasitism was estimated considering all the cells occupied by an ectoparasitoid regardless of the species.
In September 2019, we cut two branches from the upper crown of each tree. In each branch, we located and examined the shoot that had grown in the previous growing season (2018). We did not study shoots from 2019 because they were still growing when the females were laying the eggs. For each of the shoots, we counted the number of new twigs that has developed from each bud in the current (2019) growing season and the number of galls in these twigs. We used these data to calculate the ratio between the number of galls developed in the current year and the number of buds available for the wasp oviposition during that summer.
On 15 February 2020, we collected and dissected 339 buds drawn from different classes of trees (n = 84, 84, 73, and 98 buds from resistant Type I, resistant Type II, susceptible clones, and the 3 parental species, respectively; samples included 3–4 individual tree per hybrid/species and 24–28 buds per individual) to assess the presence of necrotic lesions (Figures 3A,C). Necrotic lesions were interpreted as a HR induced by the larvae (Fernandes, 1998; Ollerstam et al., 2002; Nugnes et al., 2018).
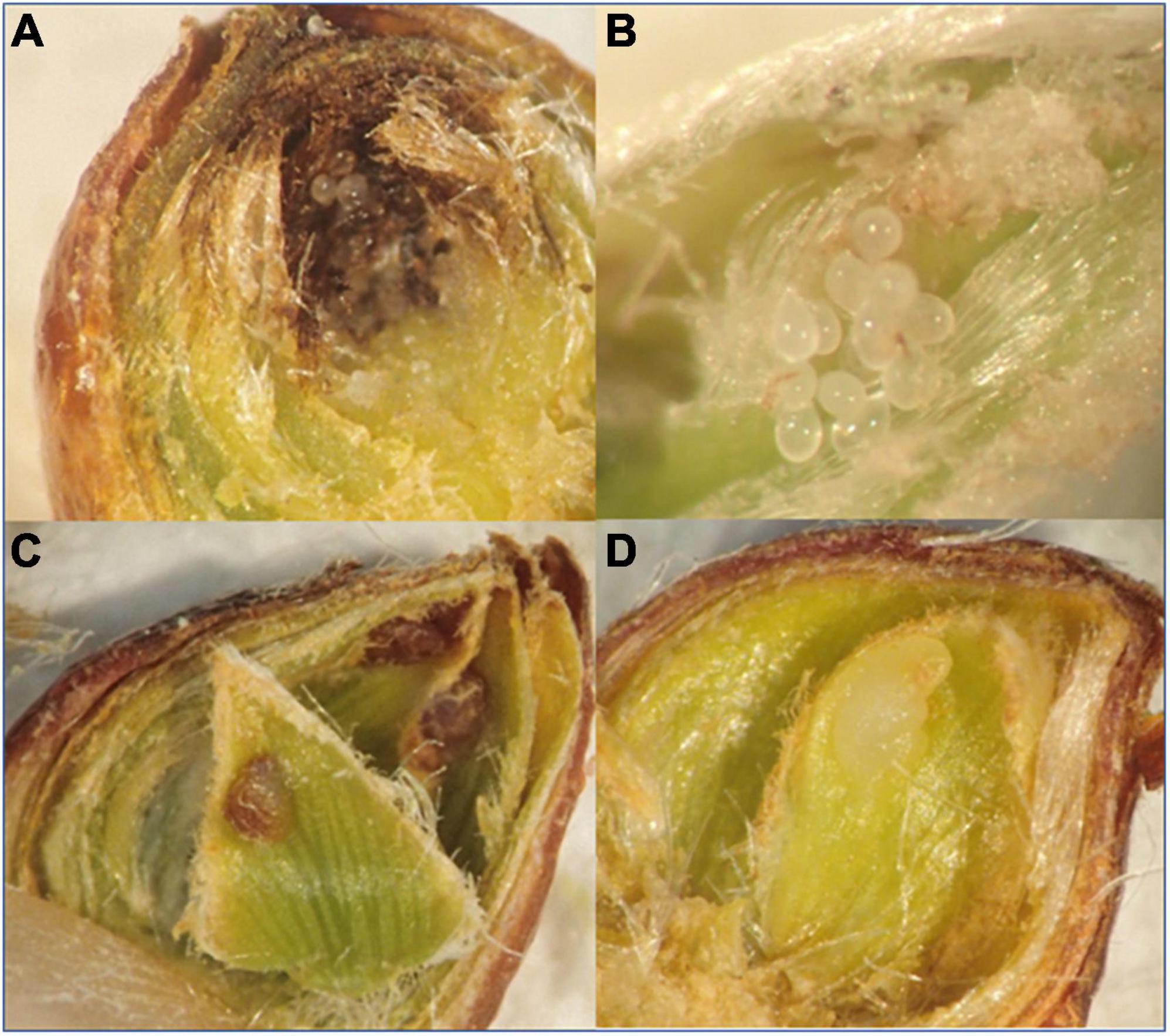
Figure 3. (A) Eggs surrounded by a necrotic lesion. (B) Dryocosmus kuriphilus eggs inside the bud. (C) Necrotic galls inside the bud. (D) Normal gall developing in leave primordium inside the bud.
At the end of August of 2020, when the female flight was over, we randomly chose three trees per clone or pure species and cut two randomly selected terminal twigs from each tree. In the laboratory, we measured the diameter of the buds in two perpendicular directions with a digital caliper (± 0.01 mm). Them we dissected the first three buds per twig under a stereomicroscope to count the number of eggs laid in each bud.
Leaf and gall phytochemistry
On 7 June 2018, at the time when galls were developing, we cut a randomly selected branch from the upper crown of each individual tree evaluated in the study to obtain fresh leaves for chemical analysis. From each cut branch, we chose five ungalled leaves and five galled leaves under attacks.
In the lab, 10 leaves per hybrid/species were used to measure leaf size, and the rest were frozen at –20°C for later analysis of nitrogen, water content, total phenols, condensed tannins, and terpenes. For each galled leaf, we analyzed separately the gall itself and the leaf tissue surrounding the gall.
To analyze water and N content, galls and leaves were weighed fresh, then oven-dried at 65°C for 48 h. The dried samples were weighed again to estimate water content, milled to a fine powder, and submitted to instant oxidation (as 0.1 g tissue samples); the gases released were identified with a conductimeter. Analyses were performed by the analytical unit of the University of Santiago de Compostela (RIAIDT).
Analysis of total terpenes followed Wainhouse et al. (1998). One gram of leaf or gall from each sample was cut in very small sections and the terpene compounds were quantitatively extracted twice with n-hexane; each extraction included 25 min in an ultrasonic bath. Later the plant material was recovered by filtration, the solvent was evaporated, and the mass of the non-volatile terpene residue was measured with a precision scale.
Total leaf phenolics were determined following Sampedro et al. (2011). Phenolics were extracted from 0.5 g of plant tissue with aqueous methanol (1:1 vol:vol) in an ultrasonic bath for 15 min, followed by centrifugation and subsequent dilution of the methanolic extract. Total phenolic content was determined colorimetrically using Folin-Ciocalteu in a BioTek Elx 850 microplate reader at 740 nm, quantified with a standard curve of tannic acid and expressed as mg tannic acid equivalents per g dry mass of plant tissue.
To analyze condensed tannins, the phenolics extract was assayed with butanol—hydrochloric acid reagent (0.7 g ferrous sulphate heptahydrate in 50 ml concentrated HCl and n-butanol added to make 1 l). The absorbance was measured at 550 nm (Waterman and Mole, 1994) and converted to mass with a standard curve from purified condensed tannins of quebracho (Schinopsis balansae Engl., Unitan Saica, Buenos Aires).
Statistical analyses
We analyzed eggs/bud (sampled in summer of 2020) with a generalized linear model (Poisson distribution and log link function) of number of eggs in each of 226 buds that were dissected: usually six buds in each of three trees from each of twelve genotypes (clones or species) within each of four classes of resistance types (species, susceptible clones, resistance Type I, and resistance Type II). The model included resistance type and genotype nested within resistance type (both as fixed effects).
Galls/bud were analyzed with a general linear model that included genotype (clones or species) as a categorical variable, buds/shoot as a continuous variable (covariate), and their interaction. This analysis was restricted to the six genotypes that displayed galls after midsummer (three species and three susceptible clones).
We analyzed the prevalence of HR with a generalized linear model (binomial distribution with logit link function) that included resistance type and clone/species nested within resistance type.
We used an ANOVA to test for patterns in the foliar chemistry of ungalled leaves among four resistance types, each represented by three clones or species. We used a two-way ANOVA to test for patterns in the foliar chemistry of three tissue types (ungalled leaf, gall, ungalled portion of galled leaf) in each of three chestnut species. Prior to analysis, measurements of terpenes, phenols, and tannins were square-root transformed to improve normality and homoscedasticity. Foliar chemistry: we refered to Figures 6, 7 show untransformed data.
We also calculate the Pearson’s correlation coefficients of the variables measured in the trees, each point representing the mean of each clone × repetition interaction.
Statistical analyses were performed with the package JMP (SAS Institute Inc.).
Results
Measurements of oviposition intensity in spring of 2020 revealed that all study trees, within all species and clones, experienced oviposition by D. kuriphilus (Figure 4A). There were modest differences in oviposition intensity among resistance types (Chi-square = 9.57, df = 3, P = 0.023), and conspicuous differences among genotypes within resistance types (Chi-square = 125.47, df = 8, P < 0.0001). Oviposition intensity was > 2 eggs/bud in C. crenata, clone ‘1483’, and clone ‘88’; other clones and species experienced similar oviposition intensity of 0.2–1.3 eggs/bud.
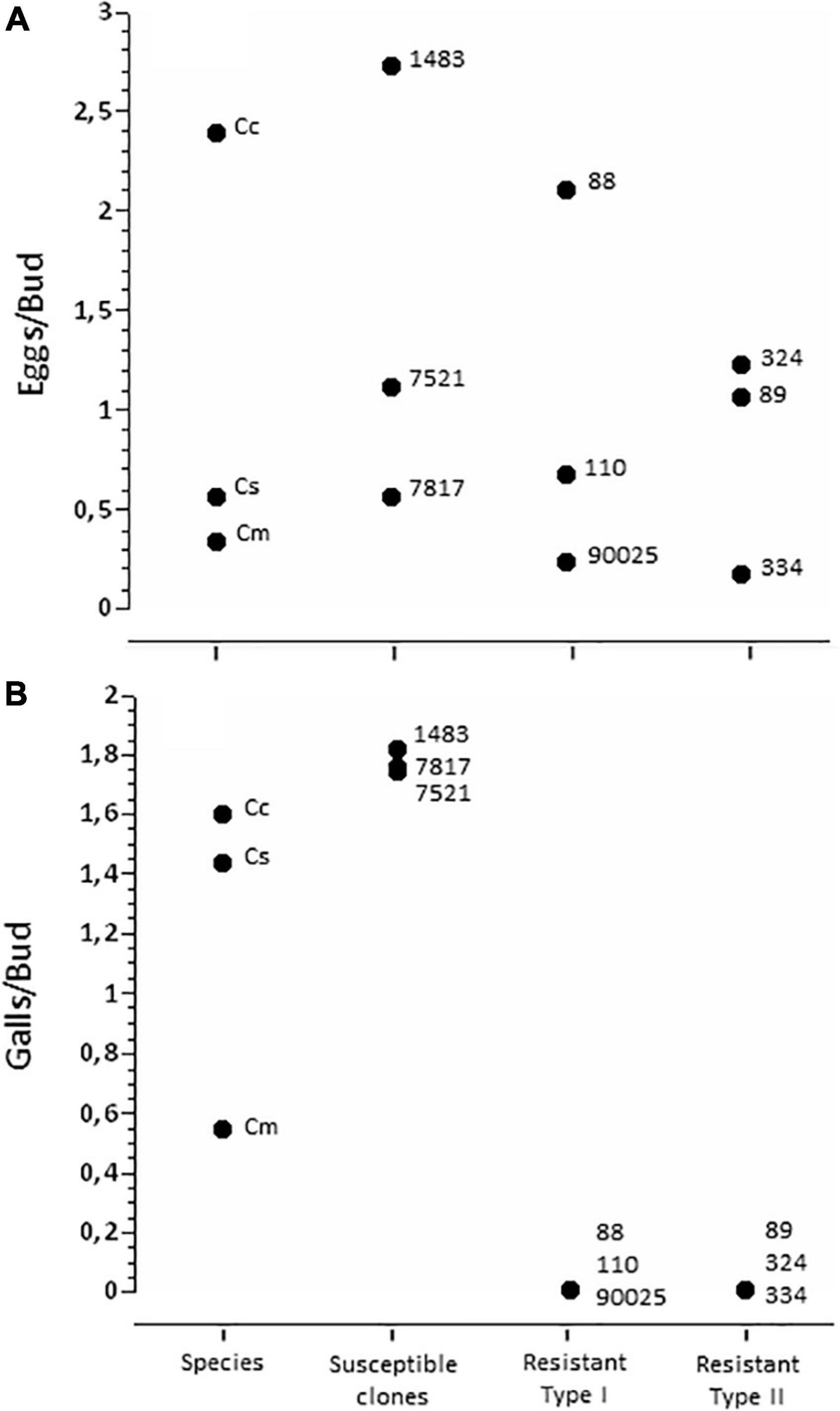
Figure 4. (A) Number of eggs per bud in the study trees. Each point represents one species or hybrid, each represented by 6 dissected buds from each of 3 trees. (B) Number of galls per bud in the study trees. Each point represents one species or hybrid, each represented by 2–8 shoots within each of 3–7 trees. Numbers show the hybrid name; Cc, Castanea crenata; Cm, C. mollissima; Cs, C. sativa.
Early in the summer, the species and three susceptible hybrid clones showed normally developed galls in buds and leaves. No galls or gall initiations were externally evident in clones classified as Type II resistance. The genotypes classified as Type I resistance, showed gall development only in the leaves (Figure 2A) and other gall initiations that did not fully form. These galls were smaller than normal galls of susceptible trees and had no more than 1 or 2 cells with living larvae inside (Figure 2A, two leaves on left). The galls were evident for only about 5–6 weeks before dropping to the soil. By midsummer, trees of Type I resistance were free of galls. The dropping galls detached from the chestnut leaves in a way consistent with leaf abscission (Figures 2C,D); the tissue around the gall was progressively detaching until there was only a hole in the leaf (Figure 2B). Many of the initial galls in clones with Type I resistance displayed no more than distorted thickening tissue (apparently a product of failed gall formation in the bud) and contained no living larvae (Figures 2E,F). Similarly deformed leaves were also present at variable densities in the rest of the study trees, resistant and susceptible.
Following the abscission of failed galls, the incidence of galls was surprisingly unrelated to oviposition intensity (Figure 4B). By the middle of the summer (2019), there were no galls in any of the trees representing clones classified as Resistant Type I or Type II. In contrast, the susceptible hybrid clones all had about 1.7 galls/bud. C. sativa and C. crenata displayed about 1.5 galls per bud in 2019; C. mollissima was lower at about 0.5 galls / bud. There were modest but significant differences in the number of galls among genotypes that displayed galls after midsummer due to the lower gall densities in C. mollissima [F(5,110) = 4.92; P = 0.0004; Figure 4B].
We observed lesions consistent with HR (Figures 3A,C) in buds of the three species, and in the resistant hybrids. The necrotic tissue was evident surrounding the eggs (Figure 3A compared with Figure 3B), and in the gall primordia that were developing inside the bud (Figure 3C compared with Figure 3D). HR (Figures 3A,C) was detectable in all hybrids and species, but were much less frequent in susceptible clones, relative to the resistant clones or the species (Figure 5; Chi-square = 53.03, df = 3, P < 0.0001). The resistant hybrids and C. mollissima showed the highest proportion of buds with HR (Figure 5), which was consistent with the lower numbers or absence of galls in these genotypes (Figure 4B).
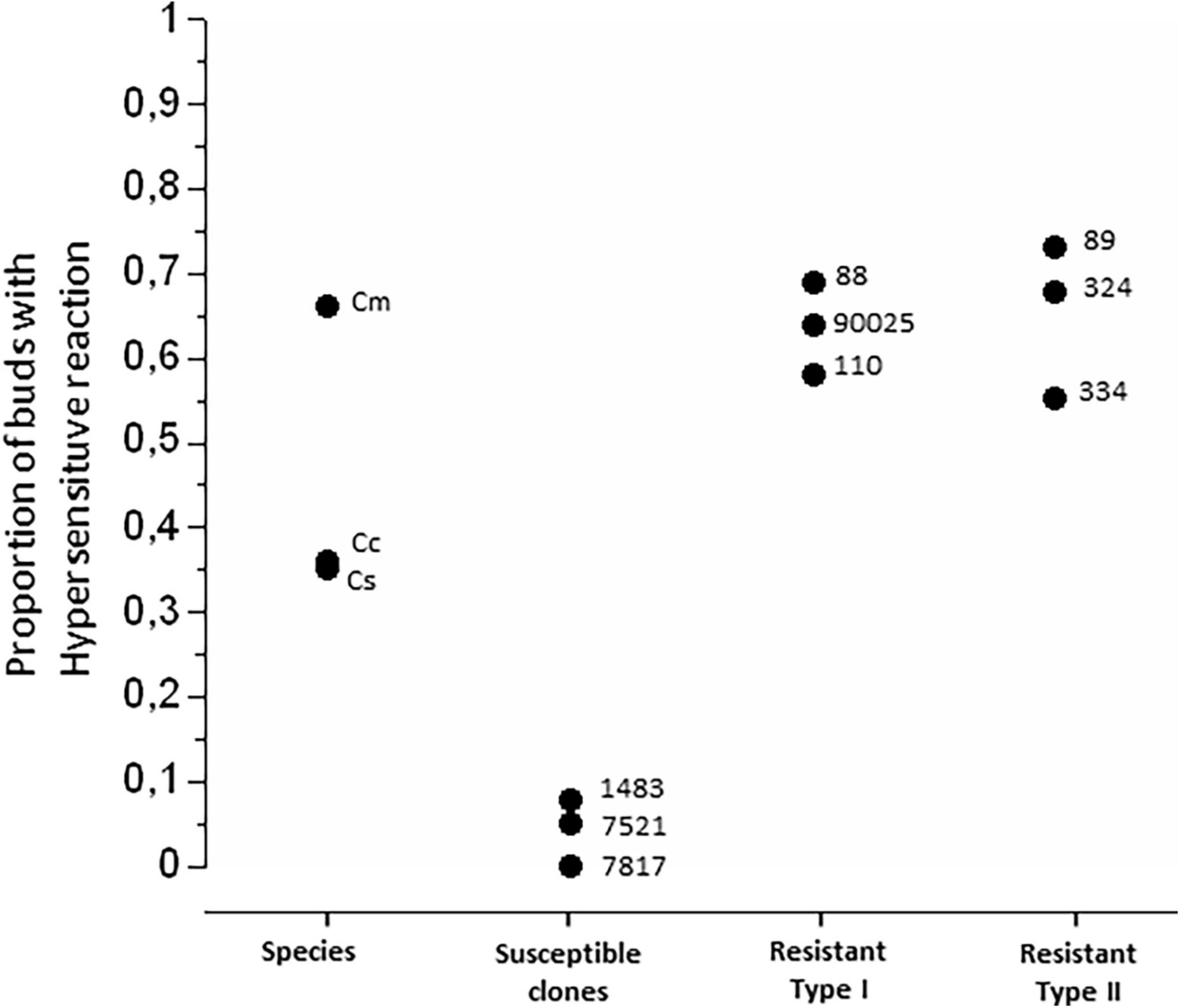
Figure 5. Proportion of buds showing necrotic lesions consistent with hypersensitive response. Each point represents one species or hybrid, each represented by dissection of about 5 buds within each of about 5 trees. Numbers show the hybrid name; Cc, Castanea crenata; Cm, C. mollissima; Cs, C. sativa.
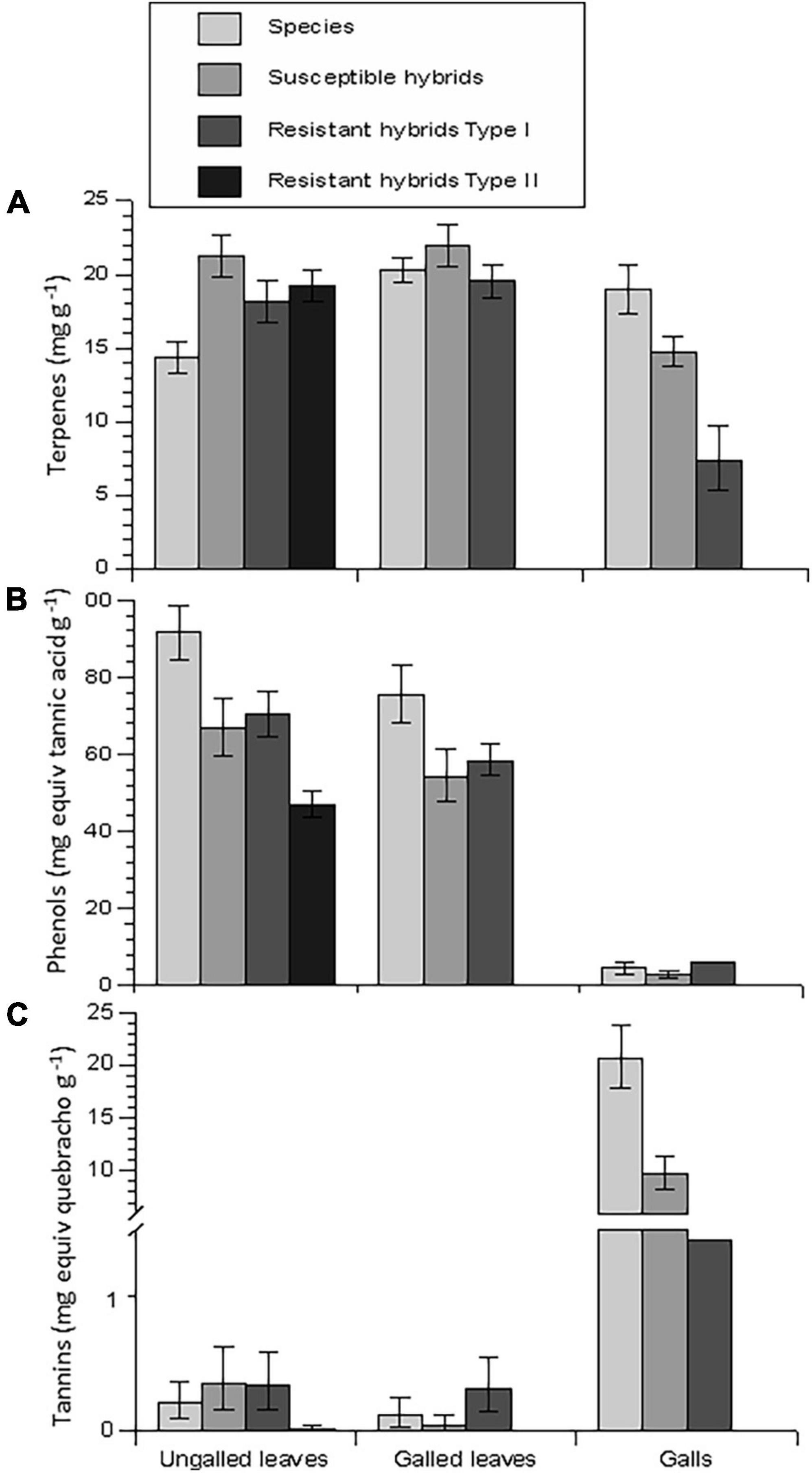
Figure 6. Foliar concentrations (A) terpenes, (B) phenolics, and (C) condensed tannins of ungalled leaves (control leaves), galled leaves, and gall tissue of the study genotypes. There was little or no gall tissue for analysis in Resistant Types I and II. Bars represent means and error bars represent standard errors.
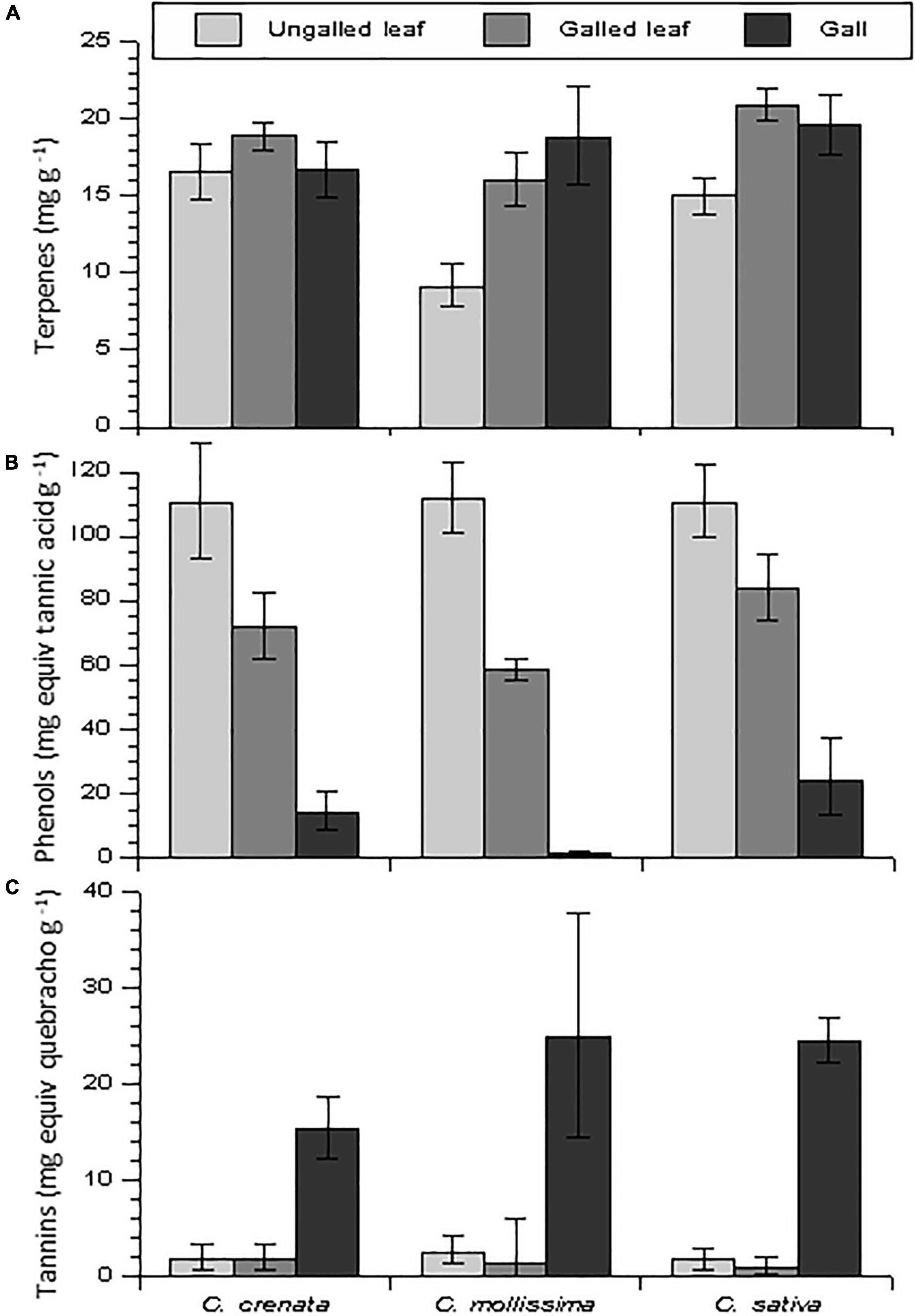
Figure 7. Foliar concentrations of (A) terpenes, (B) phenolics, and (C) condensed tannin in galled leaves, gall tissue, and ungalled leaves of three chestnut species. Bars represent means and error bars represent standard errors.
Terpene concentrations were about 15–20 mg g–1 in most genotypes and tissue types (Figures 6A, 7A). In ungalled leaves (control leaves) terpene concentrations were significantly higher in the hybrids, including the susceptible hybrids, than in the species (Figure 6A and Table 1). In C. mollissima, the species with the lowest density of galls, terpene concentrations were only about half as much in control leaves as the other two species, but increased in galls and the containing leaves to about the same as the other two species (Figure 7A and Table 2). Terpene concentrations in susceptible hybrids and hybrids of Resistance Type I were reduced by 25–50% in the actual gall tissue compared to control leaves or leaf tissue surrounding galls (Figure 6A and Table 2).

Table 1. ANOVA results testing for patterns in the foliar chemistry of ungalled leaves among four resistance types, each represented by three clones or species.
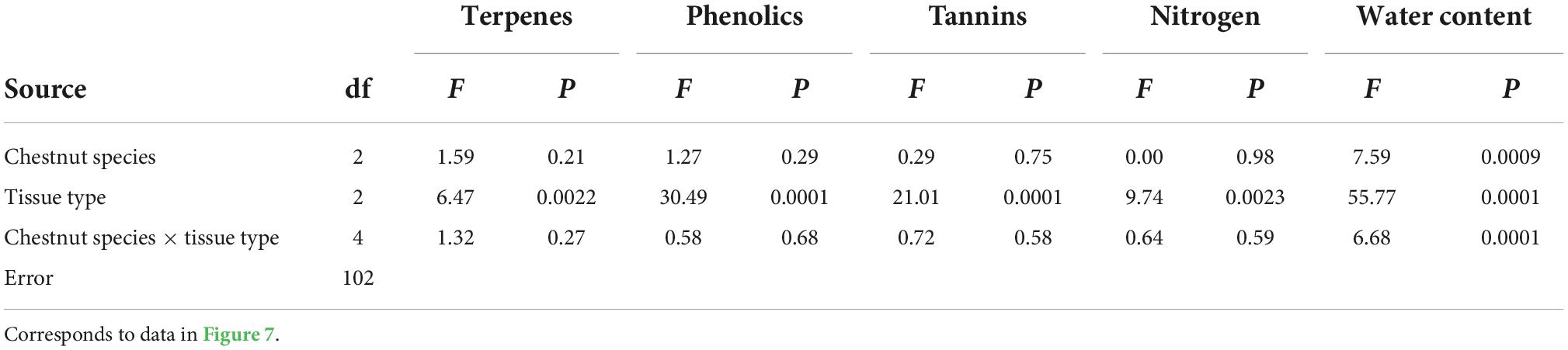
Table 2. ANOVA results testing for patterns in the foliar chemistry of three tissue types (ungalled leaf, gall, ungalled portion of galled leaf) in each of three chestnut species.
Total phenols in ungalled leaves averaged about 50–90 mg tannic acid equivalents g–1 and also differed among species and hybrids but in the opposite direction as terpenes, being higher in chestnut species than in hybrids (Figure 6B and Table 1). Phenol concentrations in gall tissue were greatly reduced (to trace levels) in all three classes of resistance types that displayed galls (Figure 6B and Table 2). There was some variation in phenol concentrations of ungalled leaves among clones within resistance classes (Table 1), but no differences among in phenol concentrations among the three species (Table 2).
Regardless of resistance type, condensed tannins were barely detectable in control leaves or leaf tissue surrounding galls (Figure 6C). However, condensed tannins in the gall tissue of species and susceptible clones were increased by about 2 orders of magnitude compared to control leaves or the leaf tissue surrounding galls (Figures 6C, 7C and Table 2). There was no variation in tannin concentration among clones within resistance type or among the three species (Figures 6C, 7C and Tables 1, 2).
Nutritional quality measured as % nitrogen in the ungalled leaves was significantly higher in hybrids than in the species, and higher in resistant than in susceptible hybrids (Figure 8A and Table 3). N concentration was elevated in gall tissue compared to ungalled leaves (about 2.75 vs. 2.55%; Figure 8A and Table 3).
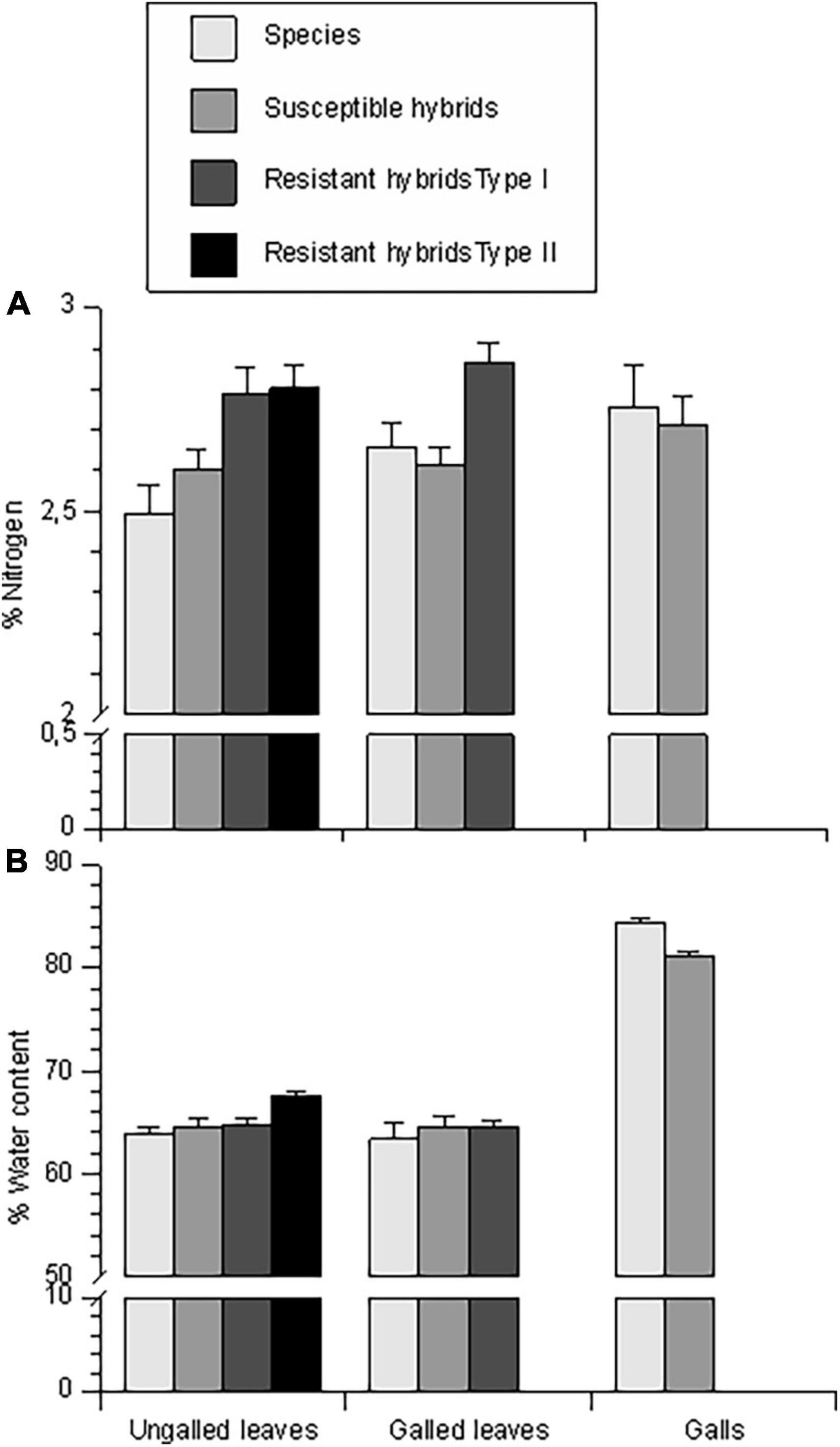
Figure 8. Foliar concentrations of nitrogen (A) and water (B) in ungalled leaves (control leaves), galled leaves, and gall tissue of the four classes of chestnut genotypes. Bars represent means and error bars represent standard errors.
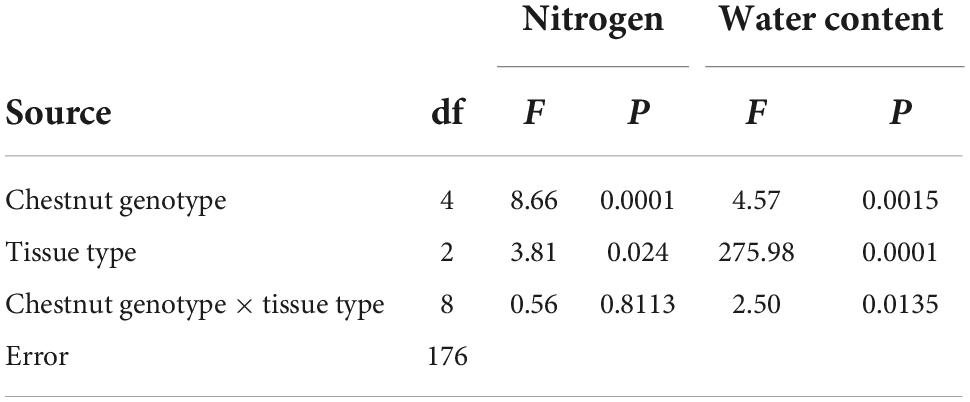
Table 3. ANOVA results testing for patterns in nitrogen and water content of three tissue types (ungalled leaf, gall, ungalled portion of galled leaf) in each of the five genotypes with galls (three species, the susceptible hybrids and resistant hybrids Type I).
Water content of ungalled leaves was similar in three of four resistance types at about 64%, but was higher, at about 66%, in clones expressing Type II resistance (Figure 8B and Table 3). Water content was much higher in gall tissue (> 80%) than in ungalled leaves or the ungalled portion of galled leaves (Figure 8B and Table 3). C. mollissima had lower water content in ungalled leaves than the other two species: mean ± SE = 60.74 ± 1.37 vs. 62.03 ± 1.32 and 62.13 ± 1.00 (Table 3).
The correlation matrix of genotype-specific attributes revealed very strong negative correlations between the frequency of HR and the frequency of galls (r < –0.90, p < 0.001; Table 4). There was a tendency for negative correlations between foliar N and gall frequency (r = –0.53 and –0.63 for correlations with total galls and galls/bud, respectively). No other measured variables showed evidence of relations with the occurrence of galls (Table 4).
Among the six genotypes that expressed galls, there were no statistically significant correlations between any measured attributes and gall volume or number of larval cells per gall (Table 5). The most suggestive pattern was a tendency for galls to have greater volume on bigger trees and those with more galls (r = 0.51–0.72; Table 5). Percent parasitism of D. kuriphilus larvae tended to be somewhat higher in genotypes that had higher terpenes in ungalled leaves, more total galls, and more galls/bud (r = 0.80, 0.82, and 0.91, respectively).
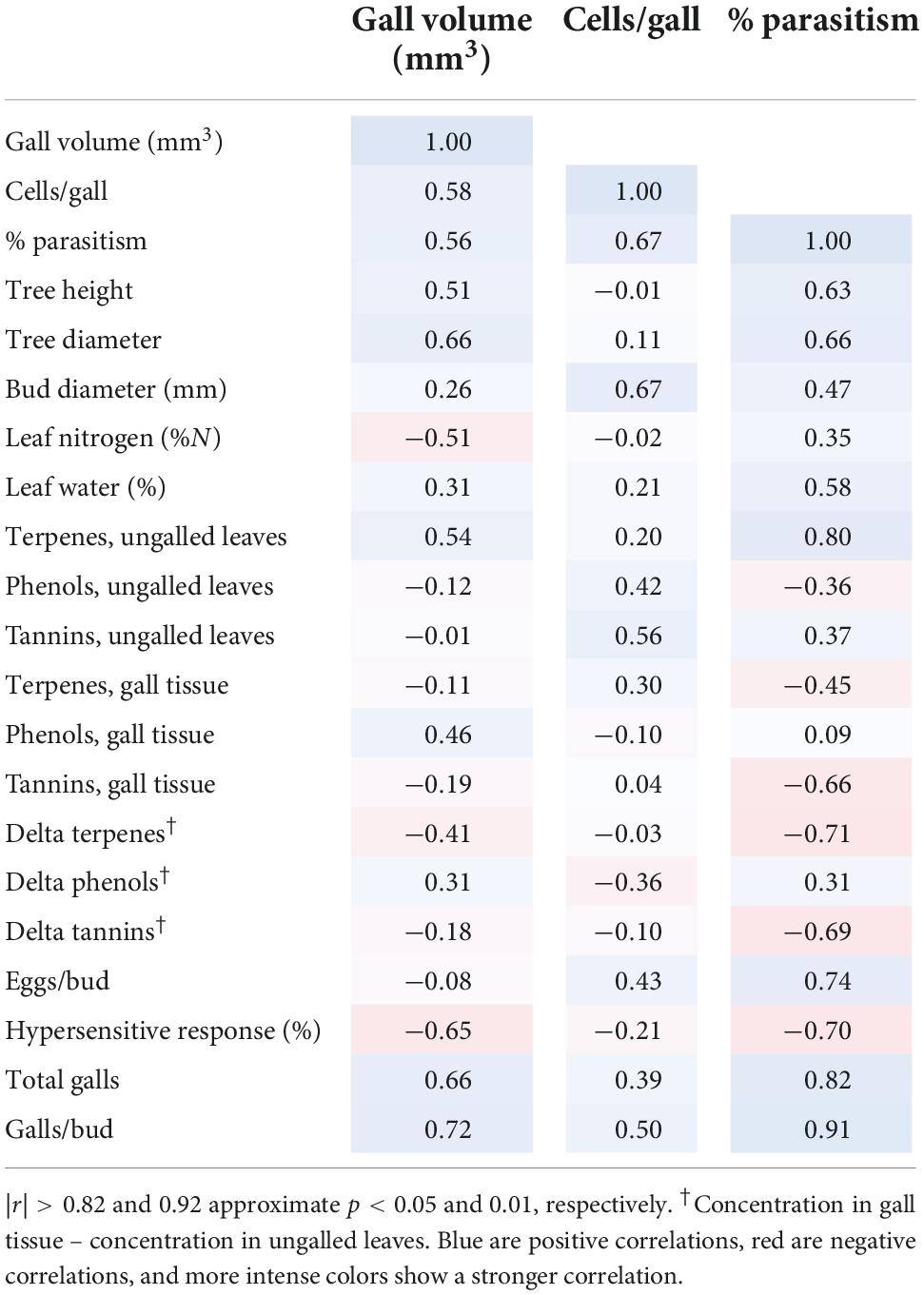
Table 5. Pearson correlations involving gall attributes for n = 6 clones that displayed normal galls.
Discussion
Host selection
There were dramatic differences among chestnut genotypes in their susceptibility to D. kuriphilus. Differences in susceptibility were not due to host selection by ovipositing females because eggs were laid freely in all the trees, resistant and susceptible. This is similar to results from Murakami (2010) for genetic varieties of C. crenata.
It was nonetheless evident that ovipositing D. kuriphilus favored some genotypes over others (Figure 4A): compared to most genotypes, there were at least 2–3 times more eggs/bud in one chestnut species (C. crenata), one clone of susceptible hybrids (‘1483’), and one clone of resistance Type I (‘88’). Later in the summer, clone ‘88’ had no galls; C. crenata and clone ‘1483’ both had high gall densities, but no higher than other genotypes that had much lower oviposition densities. Overall, the ovipositing wasps did not discriminate between hybrid host plants and their parental hosts. This differs from reports for galling insects of Quercus (Aguilar and Boecklen, 1992), Salix (Fritz et al., 1994), and Populus (Floate and Whitham, 1995). Surprisingly, D. kuriphilus did not oviposit more on the Chinese chestnut species, C. mollissima, which is its native host within most of its historical distribution.
None of the clone-specific attributes measured in this study, including plant chemistry, were associated with the oviposition preferences of wasps for chestnut trees. However, Abrahamson et al. (2003) showed that the compositions of other cynipid gall-wasp communities correlated with host plant chemistry. High concentration of terpenes (Naidoo et al., 2018) or phenolic compounds (Abrahamson et al., 2003) in plant tissue have been described as insect attractant and key stimulants for oviposition. In our study trees, there was variation in terpenes and phenols within and among hybrid genotypes and pure species, but this variation was unrelated to the number of eggs per bud. Olfactometer bioassays by Germinara et al. (2011) indicated that D. kuriphilus was attracted to olfactory cues from twigs with mechanical damage. Sevarika et al. (2021) suggested that D. kuriphilus might perceive olfactory cues, thanks to the presence of specific sensilla in the antenna. It was striking that no such associations between tree chemistry and oviposition preference were evident in our system (Table 4)—even though the adult females are ovipositing during summer when leaves are green and phytochemical cues would seem available.
The oviposition preferences of cynipid wasps have been associated with the morphology and growth vigor of host plants (Craig et al., 1989; Stone et al., 2002). We found no associations between oviposition preferences and tree size. Kato and Hijii (2001) showed that D. kuriphilus female wasps preferred large buds for oviposition. Our study trees displayed dramatic differences in bud size (0.24–4.20 mm diameter). We found no relationship between bud diameter and number of eggs, but bud diameter was positive correlated with the number of cells per gall. Each cell represents a successful larva, so this implies that larval success was somewhat greater in larger buds. However, this was a very modest effect on galling intensity compared to that from variable efficacy of inducible defenses.
Inducible defenses: Hypersensitive response
The number of wasp eggs per bud was surprisingly unrelated to the subsequent incidence of galls in various genotypes. We classified some hybrids as resistant because they displayed no galls by middle summer even after 4 years of attack. Resistant hybrids of Type I showed leaves harboring a few galls during spring; similar observations have been reported for resistant willow trees (Strong et al., 1993; Höglund et al., 2005). These differences in gall formation among genotypes may be regulated by the tree inducible response. The resistant genotypes, as well as C. mollissima, had a higher proportion of buds showing a necrotic lesion compatible with HR (Fernandes, 1998; Ollerstam et al., 2002; Figures 3A,C). It makes sense that C. mollissima showed higher HR compared with C. sativa since it has a long evolutionary history with the gall wasp. Surprisingly the resistant hybrids were crosses with C. crenata while the only hybrid involving C. mollissima (hybrid ‘7521’) was highly susceptible. Shimura (1972) reported that several cultivars of C. crenata were totally resistant to the wasp so it may be that some of the same resistant characters are in some of our hybrids.
Our most striking result was the strong negative association between HR and expression of galls: Pearson’s correlation coefficient < –0.90 with total galls and galls/bud (Table 4). HR was expressed in at least half of buds with eggs in all six resistant clones and in C. mollissima, but only about 35% in the other two species and < 10% in the susceptible clones (Figure 5). This is consistent Dini et al. (2012) who reported that the susceptible variety Madonna did no show HR. Variation in frequency and severity of HR-like necrosis within species has been previously reported (Fatouros et al., 2014; Pashalidou et al., 2015; Griese et al., 2017). Our results confirm the existence of strong genetic controls in Castanea on the strength and efficacy of HR as defenses against the eggs and neonate larvae of D. kuriphilus.
The individuals of C. sativa of our study expressed an intermediate frequency of HR (36% of attacked buds). Presumably, C. sativa would otherwise be even more susceptible to D. kuriphilus. The HR starts with molecular recognition by the plant of elicitors from the damaging agent (Iakimova et al., 2005). Because the European chestnut has no extant, native galling insects, the expression of moderate HR against D. kuriphilus must represent an ancestral trait in C. sativa. Alternatively, the elicitor could be a general molecule involved in the induction of reactive oxygen species (ROS) (Kant et al., 2015) implicated in the induction of cell death in the HR to pathogens (Lamb and Dixon, 1997).
Resistant genotypes of Type I and Type II displayed few or no galls (respectively), even if only about 60–70% of attacked buds expressed HR (Figure 5). Apparently, there are other defensive mechanisms involved and these other mechanisms are more efficient defenses in Type II hybrids compared to Type I. Some genotypes of willow that resist gall formation also showed no signs of HR (Höglund et al., 2005).
It is not clear if the HR expressed in our study material are elicited by the eggs (Viggiani and Tesone, 2009) or neonate larvae (Fukuda and Okudai, 1951; Dini et al., 2012; Nugnes et al., 2018) of D. kuriphilus. In our study area, adult oviposition begins in early June and egg hatch requires about 40 days (Avtzis et al., 2019). We observed buds with signs of HR already in August (data no shown), which was before oviposition was complete, but late enough for the first laid eggs to hatch. Therefore, HR might be elicited by products of hatching larvae (e.g., a salivary protein) or by exudates from pre-hatching eggs (Stone et al., 2002; Nugnes et al., 2018).
Two mechanisms had been proposed to explain the death of neonate larvae from HR. Reactive oxygen species (ROS) and other compounds induced during plant cell death might be acutely toxic to larvae as well as plant cells (Richael and Gilchrist, 1999). Alternatively, the HR might exert its effects less directly by interrupting nutrient supply from the plant to larvae, or simply interfering with the development of galls, which deprives them of a suitable physical habitat.
Inducible defenses: Premature abscission of attacked plant tissue
Our hybrid material classified as Type I resistance, augmented HR with targeted early abscission of attacked tissue within leaves (Figures 2C,D). Early abscission has been described as a general category of plant defenses (Boucher and Sork, 1979; Strauss and Zangerl, 2002) that can be particularly effective against insects with low mobility, such as leaf miners and gallers (Faeth et al., 1981; Stiling et al., 1991; review in Connor and Taverner, 1997). Premature abscission of herbivore-damaged leaves has been described in various forest systems including beech (Nielsen, 1978), eucalyptus (Journet, 1981), and temperate deciduous forests (Faeth et al., 1981; Bonal and Muñoz, 2008).
From the perspective of plant defense, leaf abscission can be thought of as a trade-off between the potential photosynthetic gains of the sacrificed tissue relative to what would be lost if the infesting insects were allowed to persist (Faeth et al., 1981). The relative costs and benefits for plants of leaf abscission as a defense seems likely to vary with leaf lifespan, whole-plant carbon budgets, and the precision of the tissue abscission. Hybrids in our study with Type I resistance displayed impressive precision in the abscission of insect-infested tissue. Chestnut leaves are quite large at maturity (8–22 cm length by 4–8 cm width) and were nearly as large in Type I clones following the rapid abscission of small sections of infested leaves early in leaf development (Figures 2A,B). This was qualitatively similar to many fruit trees (including almond, apricot, cherry, nectarine, peach, and plum trees) that abscise very small holes in leaves (“shot holes”) around points of pathogen infection (Sztejnberg, 1986; Ivanová et al., 2012; Zlatkovic et al., 2016).
Secondary metabolites
Our results provided no evidence that traditionally recognized classes of secondary metabolites explain variation among chestnut genotypes in resistance to the chestnut gall wasp. Constitutive levels of terpenes were actually lower in the resistant species (C. mollissima) than the susceptible species (Figure 7). C. mollissima, but not the other species, expressed an increase in terpenes in galls relative to ungalled leaves (Figure 7). Increased terpenes in galled plant tissue might have direct toxic effects on the attacking insect, as reported for galling wasps of eucalyptus (Naidoo et al., 2018), but this seems an unlikely explanation for the resistance of C. mollissima relative to the other species because even induced terpene concentrations did not exceed constitutive levels (ungalled leaves) in the other species (Figure 7). If terpene volatiles are exploited for host detection by ovipositing females, lower constitutive terpenes (as in C. mollissima) could contribute to escape from initial attacks. Total terpenes are evidently not a strong driver of oviposition preferences by D. kuriphilus: attack levels were twice as high in C. crenata as C. sativa even though they had similar terpene concentrations and C. mollissima and C. sativa had similar attack levels but different terpene concentrations (Figures 4, 7). Terpenes could also have the effect of attracting (Turlings et al., 1990; War et al., 2011) or even repelling (Rostas et al., 2013) natural enemies of the galling insect. In fact percent parasitism in our study trees was positively correlated with terpenes in ungalled leaves (Pearson’s r = 0.80, Table 4).
Phenolics and tannins have been frequently implicated as factors in the ecology of leaf-galling insects (Isaias et al., 2014), but in various ways. High levels of phenolics in host tissue have been interpreted as a defense against gall-forming insects (Tjia and Houston, 1975; Biswas et al., 2014; Otieno, 2017; Kot et al., 2018), and degradation of phenolic compounds in infested plants has been associated with increased susceptibility of host plants (Barbehenn et al., 2005; Lattanzio et al., 2006; War et al., 2012). A meta-analysis by Hall et al. (2017) found a strong general pattern of elevated tannins in the tissue of galls and their containing leaves, but they interpreted this as the result of gall-formers manipulating host chemistry to their benefit, for example by strengthening barriers to attack from their own enemies or by decreasing host suitability for their competitors (Triyogo and Yasuda, 2013). In our study trees, gall tissue had greatly elevated levels of tannins (∼10-fold) and concomitant decreases in phenolics (Figures 6, 7). The symmetry of increases in tannins and decreases in phenolics suggests that pools of phenolics were drawn down to support the biosynthesis of tannins (Seigler, 1998). Irrespective of whether the tannins and phenols function as plant defenses are a result of biochemical manipulation by D. kuriphilus, there was no evidence that they were a key determinant of genetic variation in host resistance because there was nothing distinctive about the resistant species and genotypes in the constitutive or inducible concentrations of phenols and tannins (Figures 6, 7).
Host plant manipulation
Both resistant and susceptible chestnut genotypes showed a moderate response of secondary metabolites due to the insects feeding and gall development in the leaves. Tooker et al. (2008) suggested that gallers are adapted to suppress jasmonic acid (JA, which is upregulated in plants in response to herbivore damage) because JA inhibits plant growth hormones such as auxin and cytokinins, which must be locally up-regulated in gall formation (Tooker and Helms, 2014). This may be a reason why salicylate-based defenses are prominent in the defenses of chestnuts against D. kuriphilus. It may also be relevant that salicylate-based defenses can interact antagonistically with the JA-based defenses (Walling, 2000; Thaler et al., 2012).
The most dramatic changes in chestnut phytochemistry occurred in the galls, implying that it could be a result of the insect manipulating host-plant chemistry. The larvae could benefit by the higher moisture and higher nutritional quality of the galls compared with other plant tissues (Figure 8). However, our data also showed a significant decrease of terpenes and tannins in the galls of the resistant trees compared with the susceptible hybrids (Figures 6A,C). It remains possible that the resistant genotypes were those that could be less readily manipulated by the insect. This was difficult for us to test because galls were rare or non-existent in the resistant genotypes.
Overall, our results indicate that the defense of chestnuts against D. kuriphilus relies mainly on very early HR within buds and precise abscission of nascent galls in expanding leaves. These patterns of defense are variably expressed in all genotypes but are most efficient in the resistant genotypes. The role of secondary metabolites in the defensive response of chestnuts leaves against the parasitic wasp may be less significant than in other phytophagous insects. However, this does not negate the possibility that the success of chestnut wasps also involves the suppression of plant defenses that would otherwise be induced (Strauss and Zangerl, 2002; Kant et al., 2015). The ability of gall-inducing wasps to alter plant defenses for their benefit is still poorly known.
Data availability statement
The data that support the findings of this study are available from the corresponding author upon reasonable request.
Author contributions
MA and ML conceived the idea and contributed to the data curation, formal analysis, and visualization. FC-D, PÁ-Á, and ML performed the investigation. ML wrote the original draft. All authors contributed to funding acquisition, developing methodology, reviewed and edited manuscript drafts, and approved the submitted version.
Funding
This work was supported by FEDER/Spanish Ministry of Economy and Competitiveness (Research Grant AGL2016-76262-R).
Acknowledgments
Diana Blanco, Elva Rico, Naidu Lombardero, and María Flora Romay provided assistance in the field and in the lab. Xunta de Galicia and TRAGSA provided access to the chestnut field trial.
Conflict of interest
The authors declare that the research was conducted in the absence of any commercial or financial relationships that could be construed as a potential conflict of interest.
Publisher’s note
All claims expressed in this article are solely those of the authors and do not necessarily represent those of their affiliated organizations, or those of the publisher, the editors and the reviewers. Any product that may be evaluated in this article, or claim that may be made by its manufacturer, is not guaranteed or endorsed by the publisher.
References
Abrahamson, W. G., Hunter, M. D., Melika, G., and Price, P. W. (2003). Cynipid gall-wasp communities correlate with oak chemistry. J. Chem. Ecol. 29, 208–223. doi: 10.1023/A:1021993017237
Abrahamson, W. G., Mccrea, K. D., Whitwell, A. J., and Vernieri, L. A. (1991). The role of phenolics in goldenrod ball gall resistance and formation. Biochem. Syst. Ecol. 19, 615–622. doi: 10.1016/0305-1978(91)90077-D
Aguilar, J. M., and Boecklen, J. (1992). Patterns of herbivory in the Quercus grisea x Quercus gambelii species complex. Oikos 64, 498–504. doi: 10.2307/3545167
Alcaide, F., Solla, A., Cherubini, M., Mattioni, C., Cuenca, B., Camisón, A., et al. (2020). Adaptive evolution of chestnut forests to the impact of ink disease in Spain. JSE 58, 504–516. doi: 10.1111/jse.12551
Allison, S. D., and Schultz, J. C. (2005). Biochemical responses of chestnut oak to a galling cynipid. J. Chem. Ecol. 31, 151–166. doi: 10.1007/s10886-005-0981-5
Anderson, S. S., Mccrea, K. D., Abrahamson, W. G., and Hartzel, L. M. (1989). Host genotype choice by the ball gallmaker eurosta-solidaginis (Diptera. Tephritidae). Ecology 70, 1048–1054. doi: 10.2307/1941373
Avtzis, D. N., Melika, G., Matosevic, D., and Coyle, D. R. (2019). The Asian chestnut gall wasp Dryocosmus kuriphilus: a global invader and a successful case of classical biological control. J. Pest Sci. 92, 107–115. doi: 10.1007/s10340-018-1046-1
Ayres, M. P., Clausen, T. P., MacLean, S. F., Redman, A. M., and Reichardt, P. B. (1997). Diversity of structure and antiherbivore activity in condensed tannins. Ecology 78, 1696–1712. doi: 10.1890/0012-9658(1997)078[1696:DOSAAA]2.0.CO;2
Azarkan, M., Wintjens, R., Looze, Y., and Baeyens-Volant, D. (2004). Detection of three wound-induced proteins in papaya latex. Phytochemistry 65, 525–534. doi: 10.1016/j.phytochem.2003.12.006
Bailey, R., Schonrogge, K., Cook, J. M., Melika, G., Csoka, G., Thuroczy, C., et al. (2009). Host niches and defensive extended phenotypes structure parasitoid wasp communities. PLoS. Biol. 7:e1000179. doi: 10.1371/journal.pbio.1000179
Barbehenn, R., Cheek, S., Gasperut, A., Lister, E., and Maben, R. (2005). Phenolic compounds in red oak and sugar maple leaves have prooxidant activities in the midgut fluids of Malacosoma disstria and Orgyia leucostigma caterpillars. J. Chem. Ecol. 31, 969–988. doi: 10.1007/s10886-005-4242-4
Barto, K., Enright, S., Eyles, A., Wallis, C., Chorbadjian, R., Hansen, R., et al. (2008). Effects of fertilization and fungal and insect attack on systemic protein defenses of austrian pine. J. Chem. Ecol. 34, 1392–1400. doi: 10.1007/s10886-008-9550-z
Battisti, A., Benvegnu, I., Colombari, F., and Haack, R. A. (2014). Invasion by the chestnut gall wasp in Italy causes significant yield loss in Castanea sativa nut production. Agricult. For. Entomol. 16, 75–79. doi: 10.1111/afe.12036
Bernays, E. A., Chamberlain, D., and Mccarthy, P. (1980). The differential-effects of ingested tannic-acid on different species of Acridoidea. Entomol. Exp. Appl. 28, 158–166. doi: 10.1111/j.1570-7458.1980.tb03000.x
Biswas, S. M., Chakraborty, N., and Baidyanath, P. (2014). Foliar gall and antioxidant enzyme responses in Alstonia scholaris, R. Br. after psylloid herbivory - an experimental and statistical analysis. Glob. J. Bot. Sci. 2, 12–20.
Bleiker, K. P., and Uzunovic, A. (2004). Fast- and slow-growing subalpine fir produce lesions of different sizes in response to inoculation with a blue-stain fungus associated with Dryocoetes confusus (Coleoptera : Scolytidae). Can. J. Bot. -Rev. Can. Bot. 82, 735–741. doi: 10.1139/b04-053
Bonal, R., and Muñoz, A. (2008). Seed growth suppression constrains the growth of seed parasites: premature acorn abscission reduces Curculio elephas larval size. Ecol. Entomol. 33, 31–36. doi: 10.1111/j.1365-2311.2007.00935.x
Boucher, D. H., and Sork, V. L. (1979). Early drop of nuts in response to insect infestation. Oikos 33, 440–443. doi: 10.2307/3544331
Brussino, G., Bosio, G., Baudino, M., Giordano, R., Ramello, F., and Melika, G. (2002). Pericoloso insetto esotico per il castagno europeo. L’Informatore Agrario 58, 59–62.
Castedo-Dorado, F., Alvarez-Alvarez, P., Cuenca Valera, B., and Lombardero, M. J. (2021). Local-scale dispersal patterns and susceptibility to Dryocosmus kuriphilus in different Castanea species and hybrid clones: insights from a field trial. New Forests doi: 10.1007/s11056-021-09893-8
Conedera, M., Krebs, P., Tinner, W., Pradella, M., and Torriani, D. (2004). The cultivation of Castanea sativa (Mill.) in Europe, from its origin to its diffusion on a continental scale. Veg. Hist. Archaeobot. 13, 161–179. doi: 10.1007/s00334-004-0038-7
Connor, E. F., and Taverner, M. P. (1997). The evolution and adaptive significance of the leaf-mining habit. Oikos 79, 6–25. doi: 10.2307/3546085
Cornell, H. V. (1983). The secondary chemistry and complex morphology of galls formed by the Cynipinae (Hymenoptera) - why and how. Am. Midl. Nat. 110, 225–234. doi: 10.2307/2425263
Craig, T. P., Itami, J. K., and Price, P. W. (1989). A strong relationship between oviposition preference and larval performance in a shoot-galling sawfly. Ecology 70, 1691–1699. doi: 10.2307/1938103
Dangl, J. L., and Jones, J. (2001). Plant pathogens and integrated defence responses to infection. Nature 411, 826–833. doi: 10.1038/35081161
Dini, F., Sartor, C., and Botta, R. (2012). Detection of a hypersensitive reaction in the chestnut hybrid ‘Bouche de Betizac’ infested by Dryocosmus kuriphilus Yasumatsu. Plant Physiol. Biochem. 60, 67–73. doi: 10.1016/j.plaphy.2012.07.023
Ekramoddoullah, A., Yu, X. S., Sturrock, R., Zamani, A., and Taylor, D. (2000). Detection and seasonal expression pattern of a pathogenesis-related protein (PR-10) in Douglas-fir (Pseudotsuga menziesii) tissues. Physiol. Plantarum 110, 240–247. doi: 10.1034/j.1399-3054.2000.110214.x
Eliason, E. A., and Potter, D. A. (2000). Budburst phenology, plant vigor, and host genotype effects on the leaf-galling generation of Callirhytis cornigera (Hymenoptera : Cynipidae) on pin oak. Environ. Entomol. 29, 1199–1207. doi: 10.1603/0046-225X-29.6.1199
EPPO (2005). Data sheets on quarantine pests. Dryocosmus kuriphilus. EPPO Bull. 35, 422–424. doi: 10.1111/j.1365-2338.2005.00849.x
Faeth, S. H., Connor, E. F., and Simberloff, D. (1981). Early leaf abscission - a neglected source of mortality for folivores. Am. Nat. 117, 409–415. doi: 10.1086/283724
Fatouros, N. E., Pineda, A., Huigens, M. E., Broekgaarden, C., Shimwela, M. M., Figueroa Candia, I. A., et al. (2014). Synergistic effects of direct and indirect defences on herbivore egg survival in a wild crucifer. Proc. R. Soc. B-Biol. Sci. 281:20141254. doi: 10.1098/rspb.2014.1254
Fernandes, G. W. (1990). Hypersensitivity - a neglected plant-resistance mechanism against insect herbivores. Environ. Entomol. 19, 1173–1182. doi: 10.1093/ee/19.5.1173
Fernandes, G. W. (1998). Hypersensitivity as a phenotypic basis of plant induced resistance against a galling insect (Diptera : Cecidomyiidae). Environ. Entomol. 27, 260–267. doi: 10.1093/ee/27.2.260
Fernandes, G. W. (1999). Leaflet abscission caused by a gall induced by Melaphis rhois (Homoptera: Aphididae) on Rhus glabra (Anacardiaceae). Int. J. Ecol. Environ. Sci. 25, 63–69.
Fernandes, G. W., Cornelissen, T. G., Isaias, R. M. S., and Lara, T. A. F. (2000). Plants fight gall formation: hypersensitivity. Ciência Cultura 52, 49–54.
Fernandes, G. W., Junior, P. D. M., and Schonrogge, K. (2008). Plant organ abscission and the green island effect caused by gallmidges (Cecidomyiidae) on tropical trees. Arthropod-Plant Interact. 2, 93–99. doi: 10.1007/s11829-008-9031-x
Fernandes, G. W., and Negreiros, D. (2001). The occurrence and effectiveness of hypersensitive reaction against galling herbivores across host taxa. Ecol. Entomol. 26, 46–55. doi: 10.1046/j.1365-2311.2001.00290.x
Fernandes, P., Colavolpe, M. B., Serrazina, S., and Costa, R. L. (2022). European and American chestnuts: an overview of the main threats and control efforts. Front. Plant Sci. 13:951844. doi: 10.3389/fpls.2022.951844
Fernández-López, J. (2011). Identification of the genealogy of interspecific hybrids between Castanea sativa, Castanea crenata and Castanea mollissima. For. Syst. 20, 65–80. doi: 10.5424/fs/2011201-9136
Floate, K. D., and Whitham, T. G. (1995). Insects as traits in plant systematics - their use in discriminating between hybrid cottonwoods. Can. J. Bot. -Rev. Can. Bot. 73, 1–13. doi: 10.1139/b95-001
Franceschi, V. R., Krokene, P., Christiansen, E., and Krekling, T. (2005). Anatomical and chemical defenses of conifer bark against bark beetles and other pests. New Phytol. 167, 353–375. doi: 10.1111/j.1469-8137.2005.01436.x
Freitas, T. R., Santos, J. A., Silva, A. P., and Fraga, H. (2021). Influence of climate change on chestnut trees: a review. Plants-Basel 10:1463. doi: 10.3390/plants10071463
Fritz, R. S., Nicholsorians, C. M., and Brunsfeld, S. J. (1994). Interspecific hybridization of plants and resistance to herbivores - hypotheses, genetics, and variable responses in a diverse herbivore community. Oecologia 97, 106–117. doi: 10.1007/BF00317914
Fukuda, J., and Okudai, S. (1951). Studies on the resistance of chestnut varieties to the gall wasp (Dryocosmus kuriphilus Yasumatsu). JOBAZ 16, 147–156.
Germinara, G. S., De Cristofaro, A., and Rotundo, G. (2011). Chemical cues for host location by the chestnut gall wasp. Dryocosmus kuriphilus. J. Chem. Ecol. 37, 49–56. doi: 10.1007/s10886-010-9893-0
Gil-Tapetado, D., Castedo-Dorado, F., Lombardero, M. J., Martel, J., and Álvarez-Álvarez, P. (2021). Spatial propagation and patterns of abundance of Dryocosmus kuriphilus throughout an invaded region. J. Appl. Entomol. 145, 10–25. doi: 10.1111/jen.12836
Griese, E., Dicke, M., Hilker, M., and Fatouros, N. E. (2017). Plant response to butterfly eggs: inducibility, severity and success of egg-killing leaf necrosis depends on plant genotype and egg clustering. Sci. Rep. 7:7316. doi: 10.1038/s41598-017-06704-z
Hall, C. R., Carroll, A. R., and Kitching, R. L. (2017). A meta-analysis of the effects of galling insects on host plant secondary metabolites. Arthropod-Plant Interact. 11, 463–473. doi: 10.1007/s11829-016-9486-0
Harper, L. J., Schonrogge, K., Lim, K. Y., Francis, P., and Lichtenstein, C. P. (2004). Cynipid galls: insect-induced modifications of plant development create novel plant organs. Plant Cell Environ. 27, 327–335. doi: 10.1046/j.1365-3040.2004.01145.x
Hartley, S. E. (1998). The chemical composition of plant galls: are levels of nutrients and secondary compounds controlled by the gall-former? Oecologia 113, 492–501. doi: 10.1007/s004420050401
Höglund, S., Larsson, S., and Wingsle, G. (2005). Both hypersensitive and non-hypersensitive responses are associated with resistance in Salix viminalis against the gall midge Dasineura marginemtorquens. J. Exp. Bot. 56, 3215–3222. doi: 10.1093/jxb/eri318
Iakimova, L. T., Michalczuk, L., and Woltering, E. J. (2005). Hypersensitive cell death in plants - its mechanisms and role in plant defence against pathogens. J. Fruit Ornam. Plant Res. 13, 135–158.
Inbar, M., Izhaki, I., Koplovich, A., Lupo, I., Silanikove, N., Glasser, T., et al. (2010). Why do many galls have conspicuous colors? a new hypothesis. Arthropod-Plant Interact. 4, 1–6. doi: 10.1007/s11829-009-9082-7
Isaias, R. M. S., Oliveira, D. C., Carneiro, R. G. S., Kraus, J. E., Fernandes, G. W., and Santos, J. C. (2014). “Developmental anatomy of galls in the neotropics: arthropods stimuli versus host plant constraints,” in Neotropical Insect Galls, (Dordrecht: Springer), 15–34. doi: 10.1007/978-94-017-8783-3_2
Ivanová, H., Kaloèaiová, M., and Bolvansky, M. (2012). Shot-hole disease on Prunus persica - the morphology and biology of Stigmina carpophila. Folia Oecol. 39, 21–27.
Journet, A. (1981). Insect herbivory on the australian woodland eucalypt, Eucalyptus-blakelyi M. Aust. J. Ecol. 6, 135–138. doi: 10.1111/j.1442-9993.1981.tb01283.x
Kaloshian, I. (2004). Gene-for-gene disease resistance: bridging insect pest and pathogen defense. J. Chem. Ecol. 30, 2419–2438. doi: 10.1007/s10886-004-7943-1
Kant, M. R., Jonckheere, W., Knegt, B., Lemos, F., Liu, J., Schimmel, B. C. J., et al. (2015). Mechanisms and ecological consequences of plant defence induction and suppression in herbivore communities. Ann. Bot. 115, 1015–1051. doi: 10.1093/aob/mcv054
Karban, R., and Baldwin, I. T. (1997). Induced Responses to Herbivory. Chicago, IL: University of Chicago Press. doi: 10.7208/chicago/9780226424972.001.0001
Karowe, D. N. (1989). Differential effect of tannic-acid on 2 tree-feeding lepidoptera - implications for theories of plant anti-herbivore chemistry. Oecologia 80, 507–512. doi: 10.1007/BF00380074
Kato, K., and Hijii, N. (1997). Effects of gall formation by Dryocosmus kuriphilus Yasumatsu (Hym, Cynipidae) on the growth of chestnut trees. J. Appl. Entomol. 121, 9–15. doi: 10.1111/j.1439-0418.1997.tb01363.x
Kato, K., and Hijii, N. (2001). Oviposition traits of the chestnut gall wasp Dryocosmus kuriphilus (Hymenoptera Cynipidae). Entomol. Sci. 4, 295–299.
Kessler, A., and Baldwin, I. T. (2002). Plant responses to insect herbivory: the emerging molecular analysis. Annu. Rev. Plant Biol. 53, 299–328. doi: 10.1146/annurev.arplant.53.100301.135207
Kot, I. I., Jakubczyk, A., Karas, M., and Zlotek, U. (2018). Biochemical responses induced in galls of three Cynipidae species in oak trees. Bull. Entomol. Res. 108, 494–500. doi: 10.1017/S0007485317001055
Lamb, C., and Dixon, R. A. (1997). The oxidative burst in plant disease resistance. Annu. Rev. Plant Physiol. Plant Mol. Biol. 48, 251–275. doi: 10.1146/annurev.arplant.48.1.251
Lattanzio, V., Lattanzio, V. M. T., and Cardinal, A. (2006). “Role of phenolics in the resistance mechanisms of plants against fungal pathogens and insects,” in Phytochemistry: Advances in Research, ed. F. Imperato (Trivandrum: Research Signpost), 23–67.
Lombardero, M. J., Ayres, M. P., Bonello, P., Cipollini, D., and Herms, D. A. (2016). Effects of defoliation and site quality on growth and defenses of Pinus pinaster and P. radiata. For. Ecol. Manage. 382, 39–50. doi: 10.1016/j.foreco.2016.10.003
Lombardero, M. J., Castedo-Dorado, F., and Ayres, M. P. (2021). Extreme climatic events affect populations of Asian chestnut gall wasps, Dryocosmus kuriphilus, but do not stop the spread. Agricultural Forest Entomol. 23, 473–488. doi: 10.1111/afe.12448
Marcolin, E., Pividori, M., Colombari, F., Manetti, M. C., Pelleri, F., Conedera, M., et al. (2021). Impact of the Asian gall wasp Dryocosmus kuriphilus on the radial growth of the European chestnut Castanea sativa. J. Appl. Ecol. 58, 1212–1224. doi: 10.1111/1365-2664.13861
MARM (2011). Cuarto Inventario Forestal Nacional. Dirección General del Medio Natural y Política Forestal. Madrid: Comunidad Autónoma de Galicia.
Míguez-Soto, B., Martínez-Chamorro, E., and Fernández-López, J. (2018). Tolerancia a la Avispa del Castaño (Dryocosmus kuriphilus) en Variedades Tradicionales de Fruto e Híbridos Interespecíficos. Spain: Xunta de Galicia.
Murakami, Y. (2010). “A history of studies on the chestnut gall wasp in Japan,” in A Global Serious Pest of Chestnut Trees, Dryocosmus kuriphilus: Yesterday, Today and Tomorrow, ed. S. Moriya (Japan: National Agricultural Research Center), 38–40.
Naidoo, S., Christie, N., Acosta, J. J., Mphahlele, M. M., Payn, K. G., Myburg, A. A., et al. (2018). Terpenes associated with resistance against the gall wasp, Leptocybe invasa, in Eucalyptus grandis. Plant Cell Environ. 41, 1840–1851. doi: 10.1111/pce.13323
Nielsen, B. O. (1978). Above ground food resources and herbivory in a beech forest ecosystem. Oikos 31, 273–279. doi: 10.2307/3543650
Nieves-Aldrey, J. L., Gil-Tapetado, D., Gavira, O., Boyero, J. R., Polidori, C., Lombardero, M. J., et al. (2019). Torymus sinensis Kamijo, a biocontrol agent against the invasive chestnut gall wasp Dryocosmus kuriphilus Yasumatsu in Spain: its natural dispersal from France and first data on establishment after experimental releases. For. Syst. 28:e001. doi: 10.5424/fs/2019281-14361
Nomura, M., and Itioka, T. (2002). Effects of synthesized tannin on the growth and survival of a generalist herbivorous insect, the common cutworm, Spodoptera litura Fabricius (Lepidoptera: Noctuidae). Appl. Entomol. Zool. 37, 285–289. doi: 10.1303/aez.2002.285
Nugnes, F., Gualtieri, L., Bonsignore, C. P., Parillo, R., Annarumma, R., Griffo, R., et al. (2018). Resistance of a local ecotype of Castanea sativa to Dryocosmus kuriphilus (Hymenoptera: Cynipidae) in Southern Italy. Forests 9:94. doi: 10.3390/f9020094
Nyman, T., and Julkunen-Tiitto, R. (2000). Manipulation of the phenolic chemistry of willows by gall-inducing sawflies. Proc. Natl. Acad. Sci. U S A. 97, 13184–13187. doi: 10.1073/pnas.230294097
Oliveira, D. C., Isaias, R. M. S., Fernandes, G. W., Ferreira, B. G., Carneiro, R. G. S., and Fuzaro, L. (2016). Manipulation of host plant cells and tissues by gall-inducing insects and adaptive strategies used by different feeding guilds. J. Insect Physiol. 84, 103–113. doi: 10.1016/j.jinsphys.2015.11.012
Ollerstam, O., Rohfritsch, O., Hoglund, S., and Larsson, S. (2002). A rapid hypersensitive response associated with resistance in the willow Salix viminalis against the gall midge Dasineura marginemtorquens. Entomol. Exp. Appl. 102, 153–162. doi: 10.1046/j.1570-7458.2002.00935.x
Otieno, B. A. (2017). Genetic and Environmental Influences on Utilization of Eucalyptus camaldulensis (Dehnh.) by Leptocybe invasa Fisher and LaSalle (Hymenoptera: Eulophidae). PhD thesis, Melburne, VIC: La Trobe University.
Panzavolta, T., Croci, F., Bracalini, M., Melika, G., Benedettelli, S., Tellini Florenzano, G., et al. (2018). Population dynamics of native parasitoids associated with the Asian chestnut gall wasp (Dryocosmus kuriphilus) in Italy. Psyche 2018:8078049. doi: 10.1155/2018/8078049
Park, J., and Kim, K. W. (2019). Infection scene investigation of shot holes: incomplete but neutral abscission of brown spots on oriental cherry leaves. For. Pathol. 49:e12552. doi: 10.1111/efp.12552
Pashalidou, F. G., Fatouros, N. E., Van Loon, Joop, J. A., Dicke, M., and Gols, R. (2015). Plant-mediated effects of butterfly egg deposition on subsequent caterpillar and pupal development, across different species of wild Brassicaceae. Ecol. Entomol. 40, 444–450. doi: 10.1111/een.12208
Patterson, S. E. (2001). Cutting loose. Abscission and dehiscence in Arabidopsis. Plant Physiol. 126, 494–500. doi: 10.1104/pp.126.2.494
Pearce, R. B. (1996). Antimicrobial defences in the wood of living trees. New Phytol. 132, 203–233. doi: 10.1111/j.1469-8137.1996.tb01842.x
Pires, C., and Price, P. W. (2000). Patterns of host plant growth and attack and establishment of gall-inducing wasp (Hymenoptera: Cynipidae). Environ. Entomol. 29, 49–54. doi: 10.1603/0046-225X-29.1.49
Price, P. W., Fernandes, G. W., and Waring, G. L. (1987). Adaptive nature of insect galls. Environ. Entomol. 16, 15–24. doi: 10.1093/ee/16.1.15
Quacchia, A., Moriya, S., Bosio, G., Scapin, I., and Alma, A. (2008). Rearing, release and settlement prospect in Italy of Torymus sinensis, the biological control agent of the chestnutgall wasp Dryocosmus kuriphilus. Biocontrol 53, 829–839. doi: 10.1007/s10526-007-9139-4
Quacchia, A., Ferracini, C., Nicholls, J. A., Piazza, E., Saladini, M. A., Tota, F., et al. (2013). Chalcid parasitoid community associated with the invading pest Dryocosmus kuriphilus in north-western Italy. Insect Conserv. Diver. 6, 114–123. doi: 10.1111/j.1752-4598.2012.00192.x
Rehill, B. J., and Schultz, J. C. (2012). Hormaphis hamamelidis fundatrices benefit by manipulating phenolic metabolism of their host. J. Chem. Ecol. 38, 496–498. doi: 10.1007/s10886-012-0115-9
Richael, C., and Gilchrist, D. (1999). The hypersensitive response: a case of hold or fold? Physiol. Mol. Plant Pathol. 55, 5–12. doi: 10.1006/pmpp.1999.0209
Roberts, J. A., Elliott, K. A., and Gonzalez-Carranza, Z. H. (2002). Abscission, dehiscence, and other cell separation processes. Annu. Rev. Plant Biol. 53, 131–158. doi: 10.1146/annurev.arplant.53.092701.180236
Rostas, M., Maag, D., Ikegami, M., and Inbar, M. (2013). Gall volatiles defend aphids against a browsing mammal. BMC Evol. Biol. 13:193. doi: 10.1186/1471-2148-13-193
Sampedro, L., Moreira, X., and Zas, R. (2011). Resistance and response of Pinus pinaster seedlings to Hylobius abietis after induction with methyl jasmonate. Plant Ecol. 212, 397–401. doi: 10.1007/s11258-010-9830-x
Sartor, C., Botta, R., Mellano, M. G., Beccaro, G. L., Bounous, G., Torello-Marinonia, D., et al. (2009). Evaluation of susceptibility to Dryocosmus kuriphilus Yasumatsu (Hymenoptera: Cynipidae) in Castanea sativa Miller and in hybrid cultivars. Acta Hortic. 815, 289–298. doi: 10.17660/ActaHortic.2009.815.38
Schultz, J. C. (1988). Many Factors Influence the Evolution of Herbivore Diets, but Plant Chemistry is Central. Ecology 69, 896–897. doi: 10.2307/1941239
Schultz, B. B. (1992). Insect herbivores as potential causes of mortality and adaptation in gallforming insects. Oecologia 90, 297–299. doi: 10.1007/BF00317190
Sevarika, M., Rossi Stacconi, M. V., and Romani, R. (2021). Fine morphology of antennal and ovipositor sensory structures of the gall chestnut wasp, Dryocosmus kuriphilus. Insects 12:231. doi: 10.3390/insects12030231
Shimura, I. (1972). Breeding of chestnut varieties resistant to chestnut gall wasp, Dryocosmus kuriphilus Yasumatsu. JARQ 6, 224–230.
Spoel, S. H., Johnson, J. S., and Dong, X. (2007). Regulation of tradeoffs between plant defenses against pathogens with different lifestyles. Proc. Natl. Acad. Sci. U S A. 104, 18842–18847. doi: 10.1073/pnas.0708139104
Stiling, P., Simberloff, D., and Brodbeck, B. V. (1991). Variation in rates of leaf abscission between plants may affect the distribution patterns of sessile insects. Oecologia 88, 367–370. doi: 10.1007/BF00317580
Stone, G. N., and Schönrogge, K. (2003). The adaptive significance of insect gall morphology. Trends Ecol. Evol. 18, 512–522. doi: 10.1016/S0169-5347(03)00247-7
Stone, G. N., Schonrogge, K., Atkinson, R. J., Bellido, D., and Pujade-Villar, J. (2002). The population biology of oak gall wasps (Hymenoptera : Cynipidae). Annu. Rev. Entomol. 47, 633–668. doi: 10.1146/annurev.ento.47.091201.145247
Strauss, S. Y., and Zangerl, A. R. (2002). “Plant-insect interactions in terrestrial ecosystems,” in Plant-Animal Interactions: An Evolutionary Approach, eds C. M. Herrera and O. Pellmyr (Oxford: Blackwell Science, Ltd), 77–106.
Strong, D. R., Larsson, S., and Gullberg, U. (1993). Heritability of host plant resistance to herbivory changes with gall midge density during an outbreak on willow. Evolution 47, 291–300. doi: 10.1111/j.1558-5646.1993.tb01217.x
Swapan, K. D., and Muthukrishnan, S. (1999). Pathogenesis-Related Proteins in Plants. Boca Raton, FL: CRC Press.
Sztejnberg, A. (1986). Etiology and control of cherry leaf-spot disease in israel caused by Cercospora circumscissa. Plant Dis. 70, 349–351. doi: 10.1094/PD-70-349
Taper, M. L., Zimmerman, E. M., and Case, T. J. (1986). Sources of mortality for a Cynipid Gall-Wasp (Dryocosmus dubiosus (Hymenoptera, Cynipidae)) - the importance of the tannin fungus interaction. Oecologia 68, 437–445. doi: 10.1007/BF01036752
Thaler, J. S., Humphrey, P. T., and Whiteman, N. K. (2012). Evolution of jasmonate and salicylate signal crosstalk. Trends Plant Sci. 17, 260–270. doi: 10.1016/j.tplants.2012.02.010
Tjia, B., and Houston, D. B. (1975). Phenolic constituents of norway spruce resistant or susceptible to eastern spruce gall aphid. For. Sci. 21, 180–184.
Tooker, J. F., and Helms, A. M. (2014). Phytohormone dynamics associated with gall insects, and their potential role in the evolution of the gall-inducing habit. J. Chem. Ecol. 40, 742–753. doi: 10.1007/s10886-014-0457-6
Tooker, J. F., Rohr, J. R., Abrahamson, W. G., and De Moraes, C. M. (2008). Gall insects can avoid and alter indirect plant defenses. New Phytol. 178, 657–671. doi: 10.1111/j.1469-8137.2008.02392.x
Triyogo, A., and Yasuda, H. (2013). Effect of host-plant manipulation by a gall-inducing insect on abundance of herbivores on chestnut trees. Appl. Entomol. Zool. 48, 345–353. doi: 10.1007/s13355-013-0194-2
Turlings, T., Tumlinson, J. H., and Lewis, W. J. (1990). Exploitation of herbivore-induced plant odors by host-seeking parasitic wasps. Science 250, 1251–1253. doi: 10.1126/science.250.4985.1251
Van Zandt, P. A., and Agrawal, A. A. (2004). Specificity of induced plant responses to specialist herbivores of the common milkweed Asclepias syriaca. Oikos 104, 401–409. doi: 10.1111/j.0030-1299.2004.12964.x
Viggiani, G., and Tesone, T. (2009). Phenology and monitoring of Dryocosmus kuriphilus Yasumatsu (Hymenoptera: Cynipidae) in Campania. Frustula Entomol. 32, 93–100.
Vlot, A. C., Dempsey, D. A., and Klessig, D. F. (2009). Salicylic acid, a multifaceted hormone to combat disease. Annu. Rev. Phytopathol. 47, 177–206. doi: 10.1146/annurev.phyto.050908.135202
Wagner, M. R., Clancy, K. M., Lieutier, F., and Paine, T. P. (2002). Mechanisms and Deployment of Resistance in Trees to Insects. Dordrecht: Kluwer Academic Publishers. doi: 10.1007/0-306-47596-0
Wainhouse, D., Ashburner, R., Ward, E., and Rose, J. (1998). The effect of variation in light and nitrogen on growth and defence in young Sitka Spruce. Funct. Ecol. 12, 561–572. doi: 10.1046/j.1365-2435.1998.00232.x
Walling, L. L. (2000). The myriad plant responses to herbivores. J. Plant Growth Regul. 19, 195–216. doi: 10.1007/s003440000026
War, A. R., Sharma, H. C., Paulraj, M. G., War, M. Y., and Ignacimuthu, S. (2011). Herbivore induced plant volatiles: their role in plant defense for pest management. Plant Signal. Behav. 6, 1973–1978. doi: 10.4161/psb.6.12.18053
War, A. R., Paulraj, M. G., Ahmad, T., Buhroo, A. A., Hussain, B., Ignacimuthu, S., et al. (2012). Mechanisms of plant defense against insect herbivores. Plant Signal. Behav. 7, 1306–1320. doi: 10.4161/psb.21663
Ward, H. M. (1902). On the relations between host and parasite in the Bromes and their Brown Rust, Puccinia dispersa (Erikss.). Ann. Bot. 16, 233–315. doi: 10.1093/oxfordjournals.aob.a088874
Waterman, P. G., and Mole, S. (1994). Method in Ecology. Analysis of Phenolic Plant Metabolites. London: Wiley-Blackwell.
Westphal, E., Bronner, R., and Leret, M. (1981). Changes in leaves of susceptible and resistant Solanum dulcamara infested by the gall mite Eriophyes-Cladophthirus (Acarina, Eriophyoidea). Can. J. Bot. -Rev. Can. Bot. 59, 875–882. doi: 10.1139/b81-122
Williams, A. G., and Whitham, T. G. (1986). Premature leaf abscission - an induced plant defense against gall aphids. Ecology 67, 1619–1627. doi: 10.2307/1939093
Yukawa, J. (2000). Synchronization of gallers with host plant phenology. Popul. Ecol. 42, 105–113. doi: 10.1007/PL00011989
Zhang, P., Zheng, S., van Loon, Joop, J. A., Boland, W., David, A., et al. (2009). Whiteflies interfere with indirect plant defense against spider mites in Lima bean. Proc. Natl. Acad. Sci. U S A. 106, 21202–21207. doi: 10.1073/pnas.0907890106
Keywords: Asian chestnut gall wasp, Castanea, defensive patterns, Dryocosmus kuriphilus, gall abscission, hypersensitive reaction, secondary metabolites
Citation: Lombardero MJ, Ayres MP, Álvarez-Álvarez P and Castedo-Dorado F (2022) Defensive patterns of chestnut genotypes (Castanea spp.) against the gall wasp, Dryocosmus kuriphilus. Front. For. Glob. Change 5:1046606. doi: 10.3389/ffgc.2022.1046606
Received: 16 September 2022; Accepted: 05 October 2022;
Published: 28 October 2022.
Edited by:
Alberto Santini, Institute for Sustainable Plant Protection, National Research Council (CNR), ItalyReviewed by:
Ignazio Graziosi, Innovation Pole of Genomics, Genetics and Biology (Polo GGB), ItalyChiara Ferracini, University of Turin, Italy
Copyright © 2022 Lombardero, Ayres, Álvarez-Álvarez and Castedo-Dorado. This is an open-access article distributed under the terms of the Creative Commons Attribution License (CC BY). The use, distribution or reproduction in other forums is permitted, provided the original author(s) and the copyright owner(s) are credited and that the original publication in this journal is cited, in accordance with accepted academic practice. No use, distribution or reproduction is permitted which does not comply with these terms.
*Correspondence: María J. Lombardero, mariajosefa.lombardero@usc.es